Introduction
Small box jellyfish, which belong to the family Carybdeidae of the order Cubomedusae, are small species that reside in the surface layer of marine environments with a depth of 3 m at a water temperature of 25°C. These organisms exhibit an average umbrella diameter of approximately 3 cm and are characterized by long and thin tentacles that extend up to 2 m. These jellyfish can be found in the inner bay of Namhae in Korea during the summer season.
Particle image velocimetry (PIV) is a non-contact imaging technique that enables the quantification of the hydrodynamic flow structure of aquatic organisms. PIV analyzes the velocity and structure of a flow field by tracing the pattern and center of the particles using visualization and digital image processing technology to characterize the behavior of these particles after injecting tracer particles with a specific gravity similar to the medium of the flow field. PIV minimizes external stimuli of marine organisms and enables multi-point measurements around the object. Therefore, this approach is especially well suited for analyzing the abnormal flow field of marine organisms with various shapes. Marras & Porfiri (2012) created a robotic fish and measured its flow field using PIV under non-static flow conditions. Under certain conditions, living organisms keep a certain distance from the robot, and the robot can guide living organisms. Liu et al. (2013) applied PIV to verify the results of the analysis of the unsteady flow field of a star-shaped artificial reef, and demonstrated that there is a reciprocal effect between the reefs at regular intervals. Murphy et al. (2012) quantified the flow field surrounding zooplankton by measuring the flow and swimming pattern of zooplankton with three-dimensional PIV. Flammang et al. (2011) visualized the three-dimensional vortex wake interaction between the dorsal and anal fins and tails of fish using the PIV method and found that the dorsal and anal wakes were rapidly assimilated into the caudal vortex wakes. Dabiri et al. (2010) analyzed how an optimal vortex was generated by measuring wakes with PIV in seven types of jellyfish. Lim & Demont (2009) created a mechanical crayfish model based on the kinematics of the American crayfish, visualized the abdominal flow and caudal jet with PIV, and demonstrated that it generates thrust comparable to other species with good propulsion performance. Most of the hydrodynamic forces acting on fish are caused by flow dynamics, such as changes in the fish skin and boundary layer and the size of the wake. Conversely, the kinetic reactions of fish are also largely determined by changes in flow velocity and pressure (Kim et al., 1996). Small box jellyfish swim by inhaling and expelling water internally.
Therefore, the PIV technique was used to provide accurate information about the swimming style of the small box jellyfish and to analyze the swimming style through quantitative velocity field and vorticity analysis.
Materials and Methods
As shown in Fig. 1, a total of ten specimens with umbrella diameters ranging between 2 and 4 cm were collected in Songjeong-ri (Namhae, Korea) in September 2020. To understand the swimming behavior of the small box jellyfish in the wild, they were taken to the laboratory after collection. Collected jellyfish were acclimatized for 1–2 days in a [1 m(L) × 0.5 m(W) × 1 m(H)] aquarium with circulating seawater to mimic their natural environment. The rearing water was filtered seawater under the same temperature and salinity conditions as the collected waters. As shown in Fig. 2A, the flow visualization experiment was performed using a high-speed 2,048 × 2,018 pixel camera (VH-310M-M264, ViewWorks, Anyang, Korea), a 532 nm Nd-Yag laser (VA-II-N-532-Green 5W, Viasho, Beijing, China), and PIV software (Thinkers EYES, TNTech, Busan, Korea), as well as particles (seeding particle silver coated glass spheres 20 µm, Dantec, Skovlunde, Denmark), a computer (i7, intel, Santa Clara, CA, USA), and a 500 mm (W) × 300 mm (H) × 150 mm (L) acrylic water tank. A single camera and two Nd-Yag Lasers with a beam width of 2 mm were used to scan the light source and to avoid shadows on both sides of the measurement object.
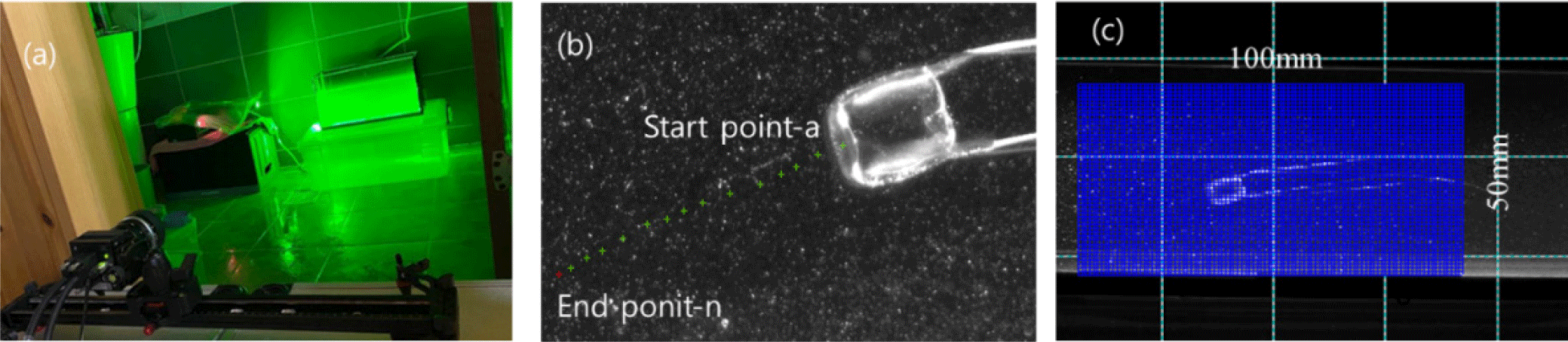
The swimming dynamics of jellyfish are characteristically asymmetric, and therefore two laser light sources with the same output were used to measure the flow on the left and right at the same time.
As shown in Fig. 2B, the internal area was calculated using the NI-Vision 2020 program (EMERSON, Seoul, Korea). The area of 14 points was measured along the outline after the image preprocessing to set the boundary through boundary point detection. The area, height, and length at 14 points were calculated as the aspect ratio. As shown in Fig. 2C, the measurement location started from 7.0 × 10–2 m from the floor and 2.0 × 10–2 m from the wall and covers an area of 5.0 × 10–2 m2 with a grid of 100 (horizontal) × 50 (vertical) pixels, and the resolution was measured to be 2.027 × 10–4 m/pixel. The PIV technique is a method for hydrodynamic analysis of the flow field that consists of injecting particles with the same specific gravity as the medium of the flow field and analyzing the behavior of these particles using digital visualization technology. PIV is a non-contact flow measurement method that is used when it is difficult to install a contact sensor and various types of flow fields. Therefore, this approach is uniquely well suited for the analysis of the behaviors of marine organisms. Simultaneous multi-point measurements provide several advantages in flow analysis around the measurement target when flow analysis of instantaneous flow changes at the measurement point is required. The formation of complex flow patterns around the jellyfish due to its long tentacles could be successfully predicted. Therefore, the swimming behavior was analyzed using simultaneous multi-point measurements.
Results and Discussion
Changes in the flow field structure were qualitatively examined and the swimming speed was measured based on quantitative data by analyzing the inhalation and discharge processes of swimming small box jellyfish using the PIV method.
The measurement reference point cannot be easily identified due to the many changes in the shape of the jellyfish induced by repeated contraction and relaxation in a short time. Therefore, the positions of 14 points and movement distances were calculated at 0.1 seconds intervals in the front of the head, where the external change was relatively small, and the results were shown in Fig. 3A. The measurements were taken from 14 points from the starting point (a) to the end point (n). The moving distance was measured at 6.371 × 10–2 m, the average moving distance was 0.46 × 10–2 m, and the average speed was 3.64 × 10–2 m/s. The maximum moving distance was calculated at 0.66 × 10–2 m and the minimum moving distance was 0.27 × 10–2 m. As shown in Fig. 3B, the maximum moving distance was 2.48 times the minimum moving distance, and the speed was proportional to this difference. The distance traveled and speed varied depending on the propulsion phase (i.e., inhalation vs. expulsion), which repeatedly decreased and increased in proportion to each other. As illustrated in Fig. 3B, the moving distance and speed decreased from point “a” to point “c,” speed increased up to point “e,” decreased up to point “h,” increased up to point “j,” decreased up to point “l,” and again increased to point “m” and then decreased thereafter. The speed for each phase was repeatedly increased and decreased at intervals of 0.15 to 0.2 seconds, and the period was measured to be approximately 0.5 seconds.
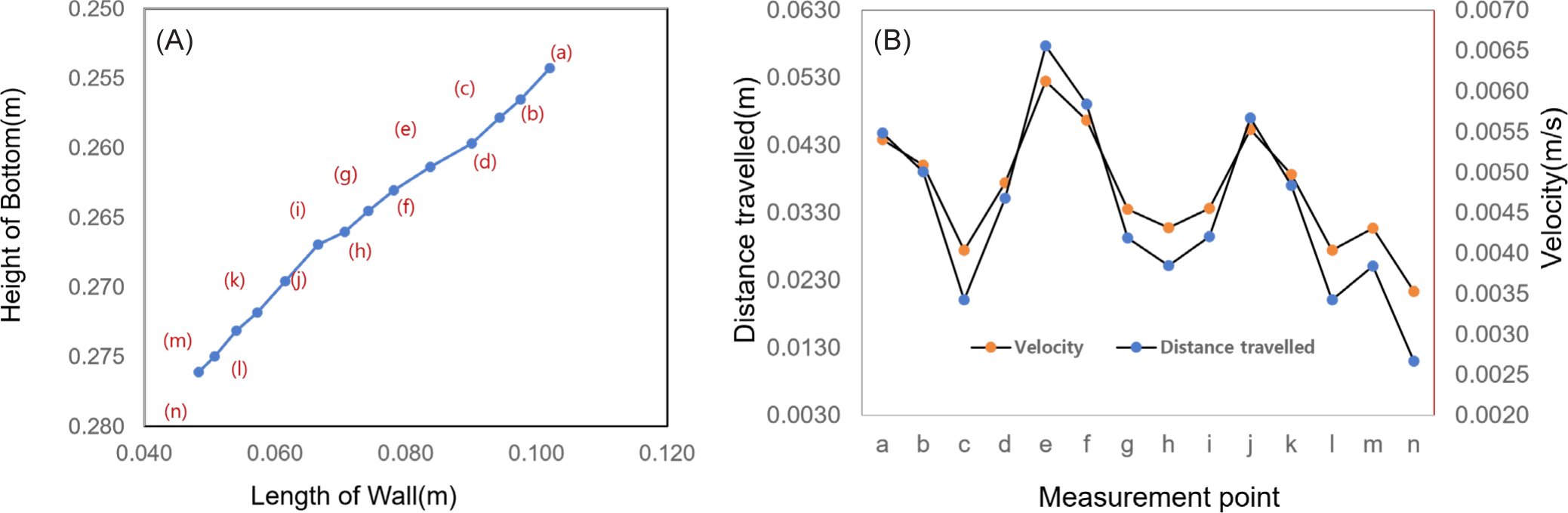
As shown in Fig. 4, the velocity distribution and streamline structure were visualized by measuring the velocity at 14 points. Since the streamline can indicate the direction of moving particles, the direction and flow rate of a fluid flowing into the body of a small box jellyfish could be predicted. The propulsion speed of the discharge part was measured to be 6.0 × 10–2 m/s, which was as high as 600% of the speed around the tentacles (1.0 × 10–2 m/s). Interestingly, this speed was proportional to the change in the internal area and the movement speed. After qualitative visualization of the flow velocity distribution and the streamline structure by analyzing the velocity field at the 14 sample points, the internal area of the small box jellyfish body was found to be consistent with the pattern of periodic increase and decrease from a minimum of 4.68 × 10–2 m2 to a maximum of 5.18 × 10–2 m2. The trailing jet of the small box jellyfish was found to have an average speed of 5.5 × 10–2 m/s, which has a propulsion capacity of 66.18% compared to the moving speed of 3.64 × 10–2 m/s. However, unlike the moon jellyfish that moves through the contraction and relaxation of its bell, the rowing pattern of the small box jellyfish exhibited no C-shaped vorticity characteristics (Kim et al., 2019). Therefore, it appeared that small box jellyfish have better swimming ability than moon jellyfish, whose swimming dynamics rely on repeated muscle contraction and relaxation.
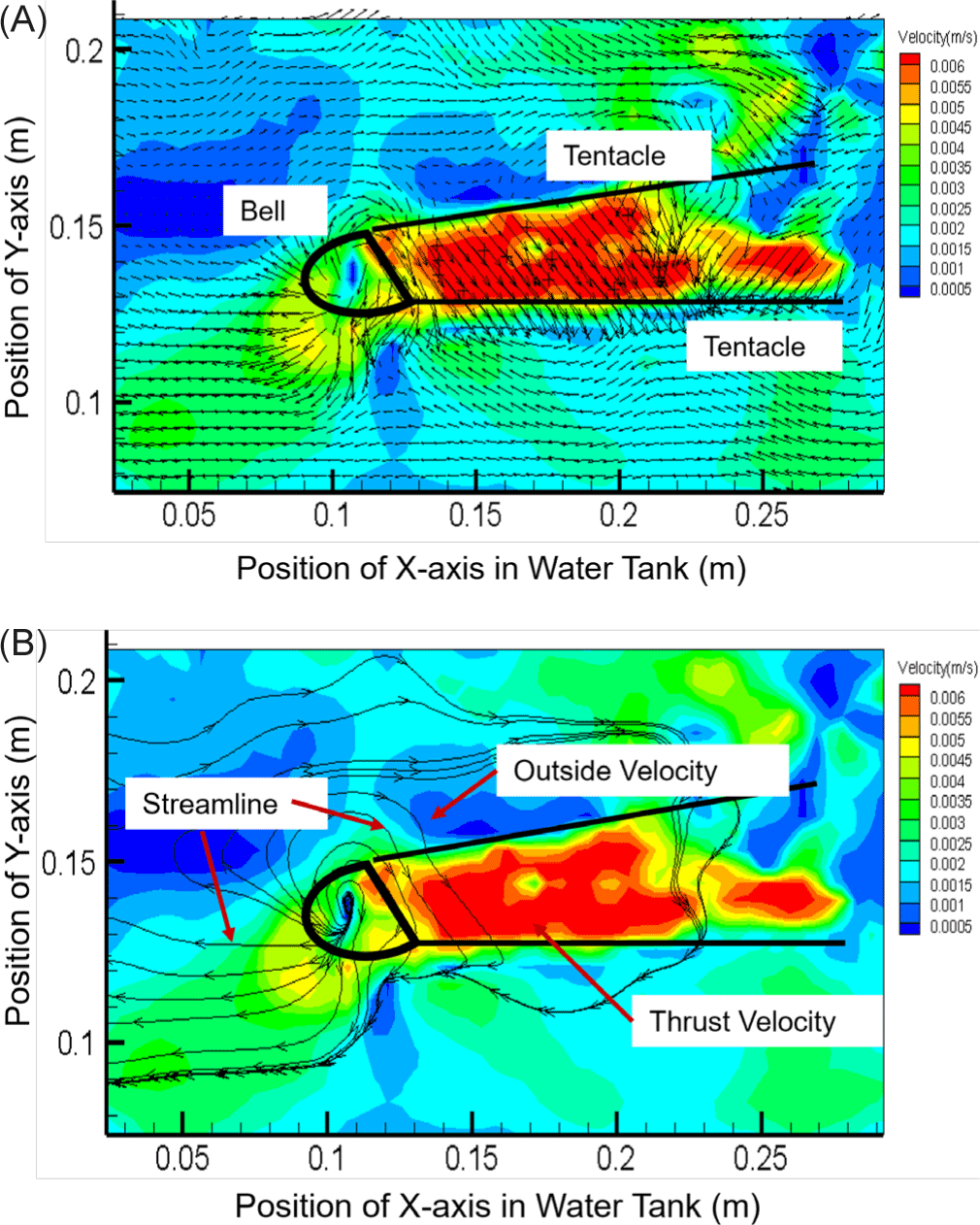
Research on mimicking marine organisms and using them for engineering purposes has been ongoing for several decades. For example, several studies (Chen et al., 2014; Fu et al., 2017; Luo et al., 2016) have reduced drag by hydro-dynamically analyzing shark skin to create a structure that can be applied to remotely operated vehicles, autonomous underwater vehicles, and swimsuits. Shen et al. (2017) fabricated four actuators by simulating the swimming behaviors of cephalopod mollusks, thus reducing energy loss and improving efficiency. Zhang et al. (2021) created a biomimetic soft robotic siphon by mimicking the siphon actuation muscles (SAMs) of cephalopods, which made it possible to increase thrust in a very short time. Szymak & Przybylski (2018) applied two fins imitating fish and seals to a biomimetic underwater vehicle and analyzed the effects of the fins. Chu et al. (2012) developed a smart actuator that mimics muscle contractions using a shape memory alloy, which achieved an increased speed per body length coupled with size and weight reduction, as well as noise reduction during the operation of a biomimetic underwater robot. As discussed above, marine organisms exhibit high-efficiency propulsion and control in water, and this ability allows them to effectively swim and control their posture in the water column while expending minimal amounts of energy. Streamlined fish like tuna show very efficient propulsion ability (Pavlov et al., 2017). Marine organisms swim in different water columns, ranging from the surface to several kilometers deep. Evolutionarily, they have a wide range of morphologies depending on the influence of fluids. The measurement of marine organisms using Marine organisms swim in different water columns, ranging from the surface to several kilometers deep. Evolutionarily, they have a wide range of morphologies depending on the influence of fluids. The measurement of marine organisms using PIV, a non-contact multipoint measurement method, provides a way to minimize external stimuli and accurately measure asymmetric swimming forms. By analyzing the quantitative velocity field and vorticity according to the appearance and swimming form of marine organisms, it is possible to identify the mechanisms of the environment and swimming form of marine organisms, and to reflect them in the placement of marine structures and the design of fishing gear.