Introduction
Seaweeds have been a dietary component and used as alternative medicine in East-asian countries such as China, Japan and Korea for several centuries. In Korea, seaweeds have been also used as a useful food resource for dry products, salt products, and condiments. Seaweed is classified into macroalgae and microalgae. Macroalgae are additionally divided into three main groups: Chlorophyceae (green algae), Rhodophyceae (red algae), and Phaeophyceae (brown algae) (Widyaswari et al., 2021). Seaweeds are known to contain a variety of biological substances with diverse health benefits (Rindi et al., 2012). Apart from the major components of seaweeds such as polysaccharides, protein, and fatty acids, they are also rich in bioactive compounds including polyphenols, peptides, sterols, flavonoids, alkaloids, and other bioactive compounds (Ahn et al., 2004; Athukorala et al., 2007; Wang et al., 2018). Especially, brown algae are known to contain bioactive compounds such as alginic acid, fucoidan, laminarin, sargassan, and to have anti-viral, anti-bacterial, anti-oxidant, anti-coagulant, anti-inflammatory, and anti-cancer activities (Kim & Jung, 2019).
Diabetes mellitus is an endocrine metabolic disorder characterized by high blood sugar levels. The long-term complications of diabetes mellitus can cause some severe symptom such as cardiovascular, nephropathy, retinopathy, and poor blood flow. Diabetes is generally classified into 2 categories; Type 1 diabetes involves T-cell mediated autoimmune destruction of the pancreatic beta-cells that produce insulin, which do not usually produce enough insulin (Simmons & Michels, 2015). Fat, liver, and muscle do not respond to insulin in type 2 diabetes, which accounts for about 90% of diabetic cases (Fan et al., 2017). The number of people living with diabetes is currently growing in worldwide and its successful treatment is not fully to be discovered (Tabish, 2007). Until now, the most effective therapeutic approach for type 2 diabetes inhibits the carbohydrate hydrolyzing enzymes, such as α-amylase and α-glucosidase in the small intestine (Gong et al., 2020). The inhibition of α-amylase and α-glucosidase can reduce the production of monosaccharides, thereby attenuating the rapid postprandial increase in blood glucose concentration. Although, acarbose and voglibose are widely used to treat against type 2 diabetes as commercialized inhibitors, they have commonly been reported to have side effects such as abdominal pain, diarrhea, and flatulence (Fujisawa et al., 2005).
Therefore, there is a need to develop the stable, reliable and safe anti-diabetes agents from natural products. Since few innovative products derived from marine are commercially available, we tried to investigate the α-amylase and α-glucosidase inhibition effects of Korean edible seaweeds to find potential antidiabetic agents in this study.
Materials and Methods
α-amylase (EC 3.2.1.1), α-glucosidase (EC 3.2.1.20), and 4-Nitrophenyl-α-D-glucopyranoside (p-NPG) were obtained from Sigma-Aldrich (St. Louis, MO, USA). Dinitrosalicylic acid was obtained from Fluka Chemicals (Madrid, Spain). Starch, sodium hydroxide (NaOH), sodium potassium tartrate, and sodium carbonate (Na2CO3) were obtained from Junsei Chemicals (Tokyo, Japan). Other chemicals and reagents were commercially available and of analytical grade.
The samples (crude extracts of 41 seaweeds) used in this study were provided by Marine Biodiversity Institute of Korea (Seocheon, Korea). The seaweeds were collected on the coast of Korea from April 2011 to June 2016. All voucher specimen were deposited at the Marine Biodiversity Institute of Korea. Briefly, each samples (41 seaweeds) were stored at –20°C after collection and lyophilized at –40°C (FDT-8650; Operon, Gimpo, Korea). After drying, the samples were grounded and stored at –20°C. Dried seaweeds were extracted three times for 1 hr each with 70% ethanol using ultrasonicator (WUC-N30H; Daihan Scientific, Seoul, Korea). Extracted samples were concentrated using a rotary evaporator (R-210; Buchi, Flawil, Switzerland) at 50°C and then the extracts were lyophilized. To obtain a stock solution of 100 mg/mL, powdered samples dissolved in dimethylsulfoxide (DMSO; Junsei Chemical, Tokyo, Japan) and stored at –70°C until used.
The α-amylase inhibition assay was performed according to the method of Kim et al. (2004) with slight modifications. 10 µL of porcine pancreatic α-amylase (EC 3.2.1.1) was incubated with sample at various concentrations. The reaction was started by adding 180 µL of 1.0% potato starch solution in 20 mM phosphate buffer (pH 6.9) to the reaction mixture. After incubation at 37.5°C for 15 min the reaction was stopped by addition of DNS (dinitro salicylic acid; 1% 3,5-dinitrosalicylic acid, 12% sodium potassium tartrate in 0.4 mol/L NaOH) color reagent solution. The mixture was then boiled for 5 min in a water bath and chilled on ice. The α-amylase-inhibitory activity was measured at 540 nm using Versamax microplate reader (Molecular Devices, California, NJ, USA). The relative inhibition was calculated using the equation:
Acarbose was tested as a positive control.
The assay for α-glycosidase inhibition was conducted by using the slightly modified method (Eom et al., 2012). 1 µL of α-glucosidase (EC 3.2.1.20) and 10 μL of each sample solution with different concentrations in 100 mmol/L potassium phosphate buffer (pH 6.8) were incubated at 37.5°C for 30 min. Then added to 4 µL of 3 mM p-NPG (Sigma-Aldrich) as a substrate. After incubation at 37°C for 30 min, mixture was added to stop reaction 100 µL of 0.1 mol/L Na2CO3. Optical density at 492 nm was measured on Versamax microplate reader (Molecular Devices). The half-maximal inhibitory concentration (IC50) value was calculated via the following equation:
Acarbose was tested as a positive control.
The inhibition kinetic study of extract on glucosidase was determined by using a Lineweaver-Burk plot which were calculated from the results according to the Michaelis-Menten kinetics at different concentrations of samples. The quantity of α-glucosidase was maintained at 1–10 μL of sample was measured with p-NPG as substrate.
Results and Discussion
The ethanolic extracts of brown, green and red seaweeds were evaluated for their α-amylase inhibitory activity compared with those of a commercial inhibitor, acarbose. The inhibitory effects on α-amylase of the brown, green, and red ethanolic extracts and acarbose are shown in Table 1. In twenty-four brown seaweeds, Ishige okamurae was the highest α-amylase inhibitory effect with IC50 value of 16.10 ± 1.01 μg/mL, followed by Ecklonia cava (30.85 ± 0.78 μg/mL), Ishige foliacea (33.68 ± 0.10 μg/mL), Desmarestia tabacoides (36.71 ± 2.91 μg/mL), Sargassum filicinum (120.69 ± 3.64 μg/mL), and Eisenia bicyclis (159.11 ± 28.79 μg/mL; Table 1). Comparing our findings with other studies showed that I. okamurae and E. cavaexhibited IC50 values of 0.30 μg/mL and 956 μg/mL (Kim, 2010; Kim et al., 2011).
Agarum cribrosum, Carpomitra costata, Dictyopteris divaricata, Distromium decumbens, Myagropsis myagroides, Padina arborescens, Padina crassaYamada, Rugulopteryx okamura, Saccharina japonica, Sargassum coreanum, Sargassum fusiforme, Sargassum horneri, Sargassum macrocarpum, Sargassum micracanthum, Sargassum miyabei Yendo, Sargassum thunbergii, Sargassum yendoi Okamura & Yamada, and Sporochnus radiciformis had no significant effects (IC50 values > 500 μg/mL) on a-amylase inhibition. Comparing these results with a recent study that M. myagroides exhibited IC50 values in the range of 2,790–4,280 μg/mL, P. arborescens and S. fusiforme exhibited IC50 values of 230 μg/mL and 153.12 μg/mL (Park & Han, 2012; Pak et al., 2015; Yang et al., 2019). S. thunbergi showed α-amylase inhibition effect (66.29%) at 5 mg/mL (Lee, 2010). The results of other studies showed that the α-amylase inhibition activities are not significantly higher in brown seaweeds as compared with these of our results.
In five green seaweeds, Caulerpa okamurae, Cladophora japonica, Cladophora wrightiana var. minor, Codium fragile, and Ulva pertusa had no significant effects (IC50 values > 500 μg/mL) on a-amylase inhibition (Table 1). Although Lordan et al. (2013) reported C. fragile showed α-amylase inhibition effect at 10 mg/mL concentration, limited information are available on α-amylase inhibitions of Korean edible green seaweed.
Twelve red seaweeds such as Acrosorium yendoi, Asparagopsis taxiformis, Callophyllis japonica, Chondracanthus tenellus, Gracilaria textorii, Gracilaria verrucosa, Grateloupia angusta, Grateloupia crispata, Grateloupia elliptica, Martensia bibarihi, Meristotheca papulosa, and Plocamium telfairiae had no significant effects (IC50 values > 500 μg/mL) on α-amylase inhibition (Table 1). Like green seaweeds, few studies identified the α-amylase inhibitions of Korean edible green seaweed. Thus, further study is needed to investigate green and red seaweeds over IC50 value of 500 μg/mL to quantify a broader spectrum of seaweeds than previously reported. Acarbose showed the α-amylase (IC50 value : 0.59 ± 0.01 μmol/L) as internal standard. As a result, the α-amylase inhibition effect of brown seaweed were consistent with these of other studies which brown seaweeds showed the strongest α-amylase inhibition than green and red seaweeds (Mikami & Hosokawa, 2013; Zaharudin et al., 2019).
The inhibitory effects on α-glucosidase of the brown, green, and red ethanolic extracts and acarbose are shown in Table 1. In brown seaweeds, I. okamuraewas the highest α-glucosidase inhibitory effect with IC50 value of 0.78 ± 0.02 μg/mL, followed by E. cava (0.87 ± 0.03 μg/mL), I. foliacea (2.51 ± 0.09 μg/mL), S. filicinum (2.96 ± 0.06 μg/mL), E. bicycls (3.57 ± 0.16 μg/mL), S. horneri (9.36 ± 0.66 μg/mL), P. crassaYamada (10.94 ± 0.70 μg/mL), S. fusiforme (39.75 ± 3.13 μg/mL), S. miyabeiYendo (80.71 ± 3.22 μg/mL), and D. divaricata (142.84 ± 50.33 μg/mL). Comparing these results with a previous study showed that E. bicyclisand exhibited IC50 values of 0.46 ± 0.00 mg/mL and I.okamurae exhibited IC50 values in the range of 220–520 μg/mL (Eom et al., 2012; Ryu et al., 2018). A. cribrosum, C. costata, D. divaricata, D. decumbens, M. myagroides, P. arborescens, R. okamurae, S. japonica,S. coreanum, S. macrocarpum, S. micracanthum, S. thunbergii, S. yendoi Okamura & Yamada, S. radiciformis had no significant effects (IC50 values > 500 μg/mL) on a-glucosidase inhibition. However, recent studies show that P. arborescensand R. okamurae exhibited IC50 values of 260 μg/mL and 50.63 µg/mL (Jeong et al., 2012; Park & Han, 2012). Like these results, the α-glucosidase inhibition activities of the S. thunbergii was 97.97% at the 0.1 mg/mL concentration (Kim et al., 2015). In green seaweeds, C. wrightiana var. minor showed the strong inhibition with IC50 value of 0.22 ± 0.01 μg/mL against α-glucosidase, followed by C. ladophora japonica (7.54 ± 0.43 μg/mL). But C. okamurae, C. fragile, and U. pertusa had no significant effects (IC50 values > 500 μg/mL) on a-glucosidase inhibition among green seaweeds. In red seaweeds, A. yendoi, C. japonica, C. tenellus, G. textorii, G. verrucosa, G. angusta, G. crispata, G. elliptica, M. bibarihi, M. papulosa, and P. telfairiae had no significant effects (IC50 values > 500 μg/mL) on a-glucosidase. Therefore, brown (I. okamurae, E. cava, I. foliacea, S. filicinum, E. bicycls, S. horneri, P. crassa Yamada, S. fusiforme, S. miyabeiYendo, and D. divaricata1), green (C. wrightiana var. minor), and red (A. taxiformis) seaweeds can be good candidates as α-glucosidase inhibitors.
Recent studies founded that the total phenolic (TP) contents (marine-derived polyphenols) are known to be have significant correlations with anti-viral, anti-bacterial, anti-cancer, anti-coagulant, anti-diabetes, anti-inflammatory, and anti-oxidant activities (Gómez-Guzmán et al., 2018; Lopes et al., 2017). Recently, TP contents of brown (range from 3.52 to 78.52 mg phloroglucinol equivalent (PGE)/g) and green (range from 2.40 to 51.87 mg PGE/g) seaweeds showed significantly higher TP content than tested red seaweeds (range from 3.24 to 33.22 mg PGE/g) (Ahmad et al., 2012; Lee et al., 2021). Therefore, the glucosidase inhibition activity of brown seaweeds may be precisely correlated with the TP contents.
Since brown seaweed such as C. wrightiana var. minor, E. cava, and I. okamurae are the strongest α-glucosidase inhibition among brown, green and red seaweeds, the Lineweaver-Burk plot analyses of C. wrightiana var. minor, E. cava, and I. okamurae were carried out to determine the type of inhibition. As shown in Fig. 1. All tested brown seaweeds were identified as non-competitive inhibitors. Thus, C. wrightiana var. minor, E. cava, and I. okamurae may bind at α-glucosidase protein sites other than the active site and cannot interact with the substrate in the active site (Blat, 2010). Our findings are in agreement with the results of Park et al. (2018), who observed E. cava ethanol extract was identified as non-competitive against α-glucosidase and I. okamurae methanol extract also reported to have a non-competitive inhibition of α-glucosidase activity. However, the α-glucosidase inhibition activity of C. wrightianavar. minor via non-competitive inhibition, which has not been reported previously.
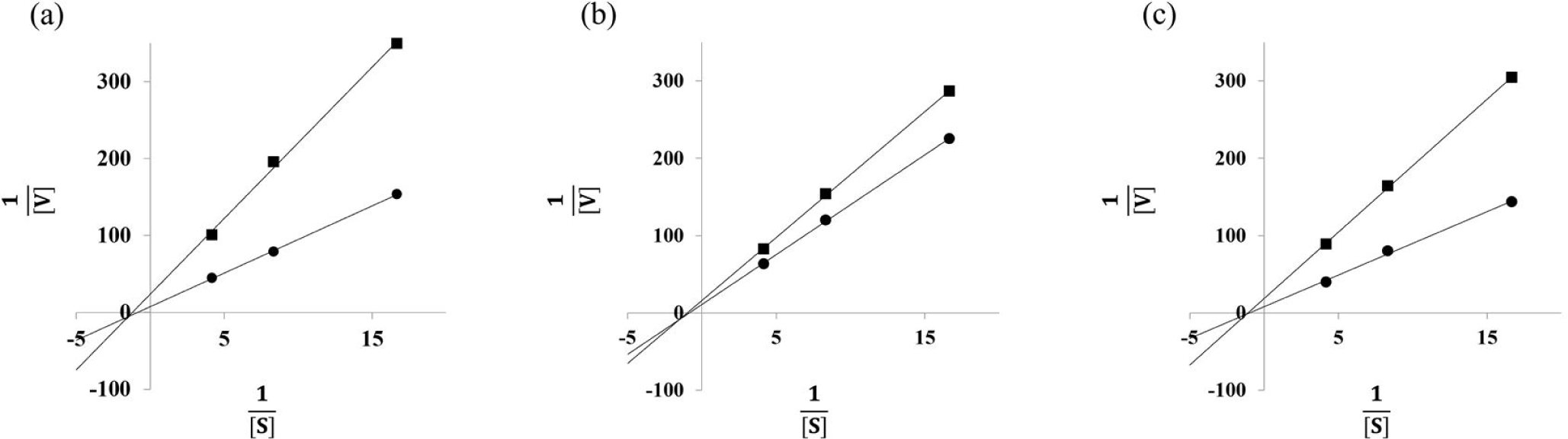
In conclusion, the assessments of α-amylase and α-glucosidase inhibition activities showed that the IC50 values in 41 samples (brown, red, and green seaweeds). E. cava, I. foliacea, and I. okamurae had high α-amylase inhibitory activities with 30.85 ± 0.78 μg/mL, 33.68 ± 0.10 μg/mL, and 16.10 ± 1.01 μg/mL of IC50values. Also, E. cava, I. foliacea, and I. okamurae had high α-glucosidase inhibitory activity with0.87 ± 0.03 μg/mL, 2.51 ± 0.09 μg/mL, and 0.78 ± 0.02 μg/mL of IC50values. While green (C. japonica IC50 = 7.54 ± 0.43 μg/mL, C. wrightiana var. minor IC50 = 0.22 ± 0.01 μg/mL) and red (A. taxiformis IC50 = 2.27 ± 0.12 μg/mL) seaweed extracts were significant effective in inhibiting α-glucosidase, they did not have any effect on inhibition of α-amylase. A. taxiformis, C. japonica, C. wrightiana var. minor, E. cava, I. foliacea, and I. okamuraewere presumed to have anti-diabetes effects via inhibition on α-glucosidase among 41 seaweeds. In kinetic study, C. wrightiana var. minor, E. cava, and I. okamuraehave known as non-competitive inhibition patterns. Therefore, E. cava, I. foliacea, and I. okamurae can be good candidate as a α-glucosidase inhibitor.