Background
In marine organisms, environmental changes in the water cause a variety of physiological stress responses, such as changes in oxidative stress, decline in immunity, and changes in plasma components. Among these responses, changes in the immune system are particularly important in the context of fish disease outbreaks in the face of environmental change. Physical factors, such as extreme salinity and temperature changes, affect growth, metabolism, osmoregulation reproductive function, and immune function (Ackerman et al. 2000; Borski et al. 1994; Sampaio and Bianchini 2002; Bly and Clem 1992; Bowden 2008; Schreck et al. 1989; Britoa et al. 2000). Furthermore, stress induced by changes in salinity and temperature has been associated with enhanced generation of reactive oxygen species (ROS) in fish, which may seriously affect their immune function and antioxidant defense abilities (Paital and Chainy 2010; An et al. 2010; Kim and Kang 2015).
Phosphoinositide-specific phospholipase C (PLC) enzymes have been shown to play a role in immune responses in mammals; however, little is known about their function in marine organisms in response to stress. PLC is a family of enzymes that hydrolyzes the inner membrane element phosphatidylinositol-4,5-bisphosphate (PIP2) to produce inositol 1,4,5-trisphosphate (IP3) and diacylglycerol (DAG) (Rhee 2001). IP3 binds to IP3-specific receptors on the membrane of the endoplasmic reticulum (ER), inducing the release of Ca2+ level from the intracellular cytoplasm. While DAG initiates the activation of Ras proteins along with this Ca2+ release, which in turn activates protein kinase C (PKC) (Bunney and Katan 2011). Thirteen PLC isozymes have been cloned from mammalian species to date and have been classified into six classes according to their structures and mechanism of actions: PLC-β (1–4), PLC-γ (1 and 2), PLC-δ (1, 3, and 4), PLC-ε, PLC-ζ, and the recently identified PLC-η (1 and 2) (Rebecchi and Pentyala 2000). The PLC-β subfamily (1–4) has a significantly conserved structure of an N-terminal pleckstrin homology (PH) domain, four EF hand repeats, a triose phosphate isomerase (TIM)-like barrel domain split into an X and Y catalytic domain, a C2 domain, and a C-terminal (CT) domain (Otaegui et al. 2010). The PH domain plays a minor role in association to the membrane (Tall et al. 1997), whereas its most important role is in mediating protein-protein interactions. The EF hand repeats support the loop related to stimulation of GTP hydrolysis. The catalytic X and Y domains are necessary for the catalysis of PIP2 to IP3 and DAG (Song et al. 2001). The C2 domain serves as an intra- and intermolecular regulatory binding position, and the CT domain, the most unique feature, interacts with the GTP-bound α subunit of heterotrimeric G proteins to stimulate PLC-β (Singer et al. 2002).
The identified signaling molecules of PLCs have also been suggested to perform a role in the regulation of chemokine-mediated cell migration. There is substantial evidence suggesting a role of PLC-β in the immune response. PLC-β activity is started by G protein coupled receptor (GPCR)-mediated signal pathway (Kim et al. 2011). PLC-β plays an essential role in T cell chemotaxis (Bach et al. 2007) and has previously been shown to participate in several aspects of lymphocyte function, including cell proliferation, rescue from apoptosis, and CD4+ and CD8+ T cell differentiation (Sasaki et al. 2000; Ward and Cantrell 2001).
The stimulatory effects of lipopolysaccharide (LPS), concanavalin A (ConA), and polyinosinic:polycytidylic acid (PolyI:C) induced the activity of PKC and other mitogens. Previous studies have shown that LPS, ConA, and PolyI:C stimulation induced phosphoinositide-specific PLC (PI-PLC) and phosphatidylcholine-specific PLC (PC-PLC) expression of the kidney and spleen. (Chen et al. 1998; Wang et al. 1998; Schütze et al. 1992). Thus, the stimulatory effect contributes to both the innate and adaptive immune responses. However, unlike the signaling pathways of mammalian PLC isoforms, the intra and intercellular signaling pathways of marine organisms remain relatively elusive.
In particular, at the moment, only a few studies have examined the function of PLC-β1 in response to stress challenge. Here, therefore, we evaluated changes in PLC-β1 expression in response to external stress, elicited by pathogen-associated molecular pathogen (PAMP) challenge and environmental challenge (temperature and salinity) in the olive flounder (Paralichthys olivaceus).
Methods
LPS is a major component of the gram-negative cell wall. ConA triggers a release mechanism similar to that elicited by the specific worm allergen (Keller 1973), and PolyI:C is structurally similar to a double-stranded RNA virus and potent inducer of interferon (IFN) (Akiyama et al. 1985; Manetti et al. 1995). To study the immune response of PLC-β1 in the olive flounder (P. olivaceus), we performed immune challenge experiments by using commercially available LPS (Sigma), ConA (Sigma), and PolyI:C (Sigma). Healthy juvenile P. olivaceus (40 ± 12 g) were anesthetized with MS-222 (3-aminobenzoic acid ethyl ester; Sigma, USA) and were intraperitoneally injected with 500 μl of LPS (100 μg/ml), ConA (100 μg/ml), PolyI:C (100 μg/ml), and PBS (500 μl; as a control), as per the body mass of the fish. Target tissues were removed from P. olivaceus at 0, 1, 3, 6, and 24 h after injection. All procedures were performed according to the American Veterinary Medical Association guidelines on euthanasia.
P. olivaceus with a mean weight of 32 ± 7 g were obtained from a commercial fish farm. The fish were conditioned in aerated 200-l tanks at 20 °C for a week prior to experimentation. During the acclimatization, the fish were fed with a commercial pelleted diet twice a day (at 10:00 and 17:00). Half of the water (30‰ ± 0.2) in the tank was changed every day. Salinity was decreased at 5‰ per hour by adding fresh water (Bio Safe 50 ml/200 l water) and was regulated from 30‰ to zero. The water temperature was rapidly increased from 20 to 30 °C at a rate of 2.5 °C/h. The brain, gill, heart, liver, spleen, stomach, intestine, kidney, and muscle tissue were analyzed after 24 h. No mortality was observed following injection and environmental stress.
The total RNA was isolated using the TRIzol® kit (Invitrogen), according to the method described by the manufacturer. Complementary DNA (cDNA) was synthesized from this isolated mRNA by using the Transcriptor First Strand cDNA Synthesis Kit (Roche) and then was used as the template for amplification. Purified RNA was quantified on the basis of its optical density at 260 nm by using a UV spectrophotometer (Ultrospec 6300 pro, Amersham Biosciences). Two micrograms of total RNA was reverse-transcribed with an oligo (dT)18 and random hexamer primers and Superscript™ III reverse transcriptase (Invitrogen, USA), as per the manufacturer’s instruction. Reverse transcription was carried out at 42 °C for 60 min.
In order to analyze the tissue expression of the PoPLC-β1 mRNA, reverse transcription (RT)-PCR was performed. The 18SrRNA and β-actin genes of P. olivaceus were used as internal controls. All the PCR cycles were performed as follows: 94 °C for 5 min, 25–30 cycles (25 cycles for 18SrRNA and β-actin; 30 cycles for PoPLC-β1) of 94 °C for 30 s, 58 °C for 30 s, 72 °C for 20 s, and a final 7-min elongation step at 72 °C. The amplified PCR products were separated on 1.0 % agarose/TAE gels containing ethidium bromide and visualized with a Gel Doc image analysis system (Bio-Rad, USA). The resultant products were purified via agarose gel extraction (QIAquick® Gel Extraction kit) and sequenced (COSMO Co. Ltd., DNA Sequencing Service, Seoul, Korea).
Total RNA from various tissues was prepared as per the previously described method. 18SrRNA from a constitutive expression gene of β-actin was used as the internal control to verify the real-time PCR reaction. Quantitative real-time PCR for the tissue-specific expression analysis of PoPLC-β1 with the gene-specific primers was conducted using a LightCycler® 480 II SYBR Green (Roche, Switzerland). The SYBR Green RT-PCR assay was performed by the method described previously (Guan et al. 2007) using the ΔΔCt method, and the relative quantitative values were expressed in accordance with the 2−ΔΔCt method. In addition to investigating stimulation, the experiments also analyzed the expression of interleukins 1β and 6, as they are a lymphocyte mitogen and proinflammatory cytokines (Benveniste 1998).
Results
After confirming the sequence of PoPLC-β1 with those previously published sequences (Seo et al. 2011), we compared the characteristic regions of PoPLC-β1. The deduced product was examined using a specific primer for RT-PCR and quantitative real-time PCR (Table 1).
The expression pattern of the PoPLC-β1 gene in the brain, eye, gills, heart, esophagus, liver, spleen, pyloric ceca, stomach, intestine, kidney, and muscle was analyzed by RT-PCR and real-time PCR. All tissues showed detectable levels of PoPLC-β1 mRNA expression, but expression was especially higher in the brain and heart tissues (Fig. 1).
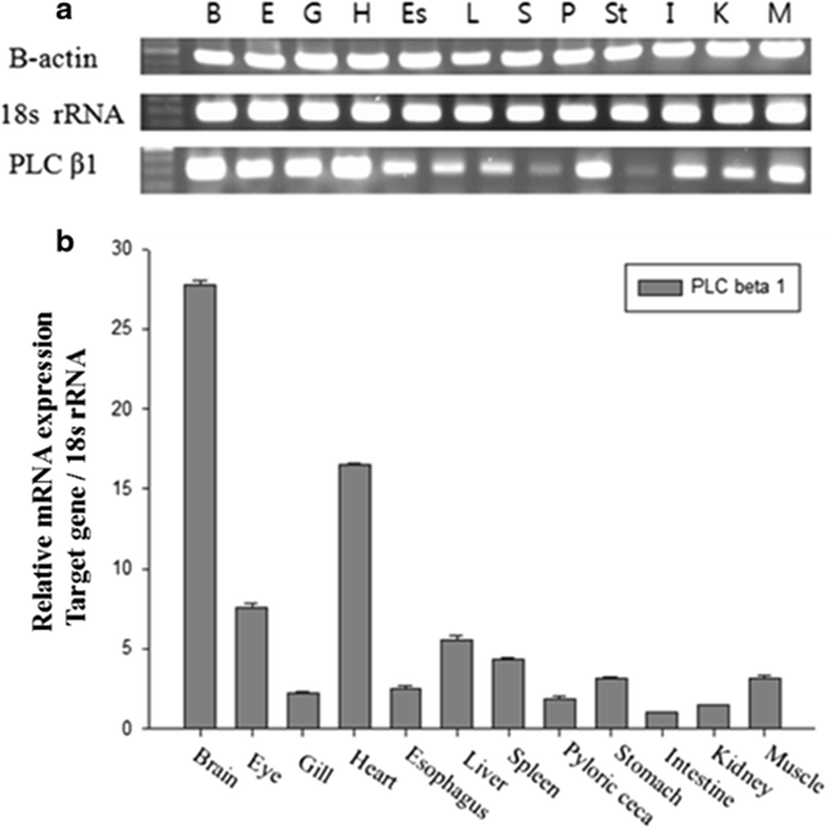
In mammals, G protein has been shown to modulate the signal transduction of both PLC-β isoforms (Charo et al. 1994; Franci et al. 1995). To evaluate general changes in the expression levels of PLC-β1 in P. olivaceus related to the immune response, we used RT-PCR and real-time PCR to compare PoPLC-β1 expression to the interleukin gene expression profiles (IL-1β, IL-6) in the spleen and kidney, following stimulation of LPS, ConA, and PolyI:C, which play a major role in various disease processes in teleost fish (Figs. 2, 3, and 4).
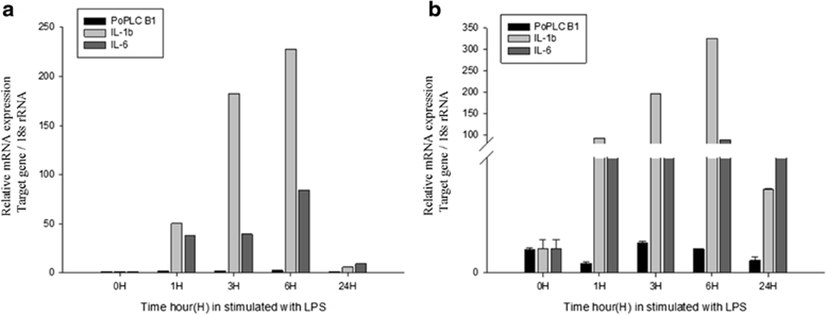
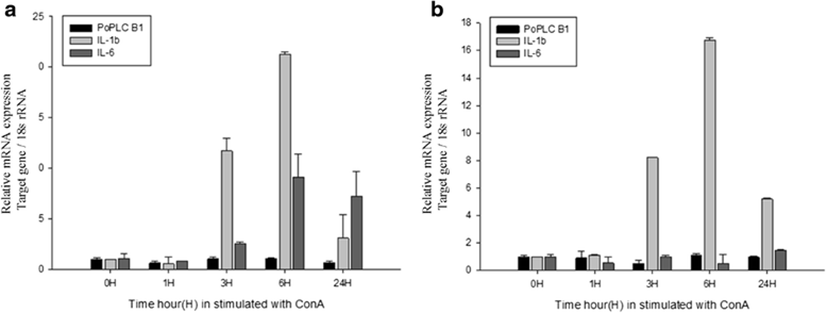
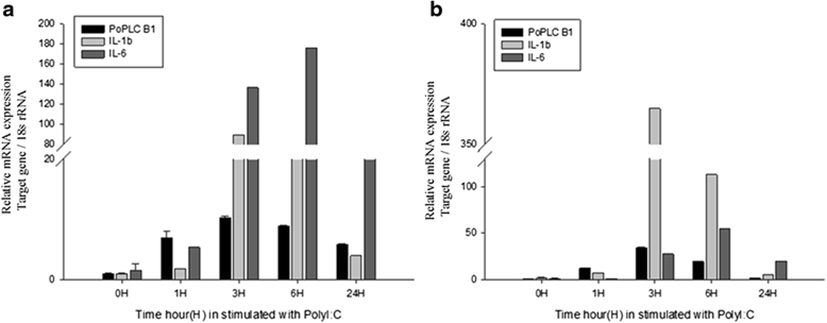
LPS and ConA stimulation did not induce significant changes in the expression level of PoPLC-β1 (Figs. 5 and 6). However, PolyI:C injection in spleen and kidney led to a dramatic increase in the expression of PoPLC-β1 at 3 h post injection, which was increased by more than 10-fold compared to the control groups and a time dependent increase was observed until 24 h post injection in the spleen and kidney (Fig. 7). As shown in Figs. 4 and 7, PoPLC-β1 expression significantly increased along with the IL-1β and IL-6 expression in the spleen and kidney following PolyI:C stimulation. The mRNA levels of IL-1β and IL-6 in the infected spleen and kidney tissues were sharply increased and reached a peak at 3 or 6 h post injection.
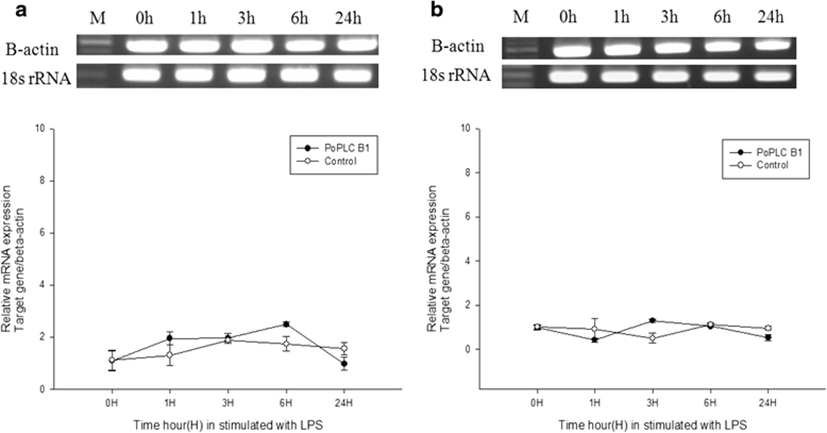
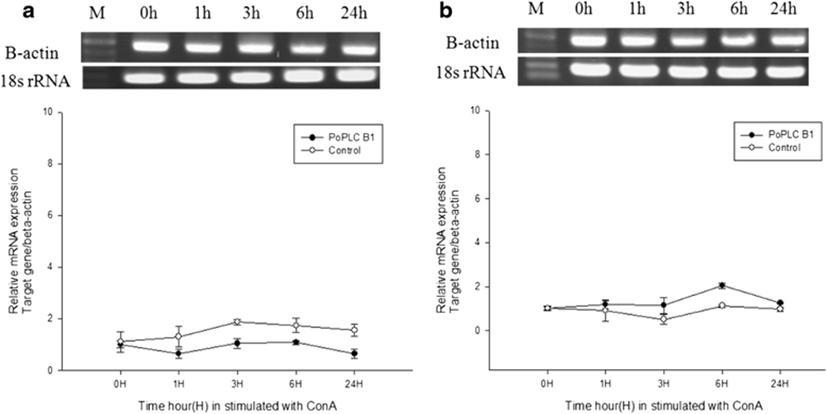
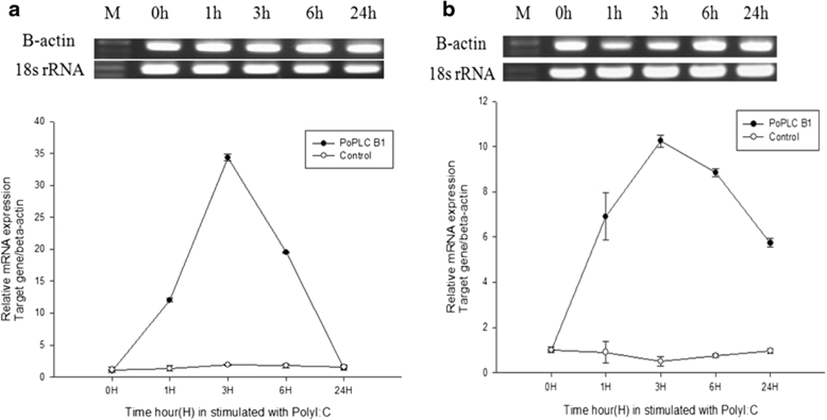
Changes of water temperature and salinity both affected the expression of PoPLC-β1. To our surprise, salinity stress induced a greater effect than thermal stress. In the salinity stress condition, the expression level of PoPLC-β1 was increased by twofold in both the brain and heart and was slightly increased in the gills (Fig. 8a). Under temperature stress, PoPLC-β1 expression increased by twofold in the heart compared to controls (Fig. 8b).
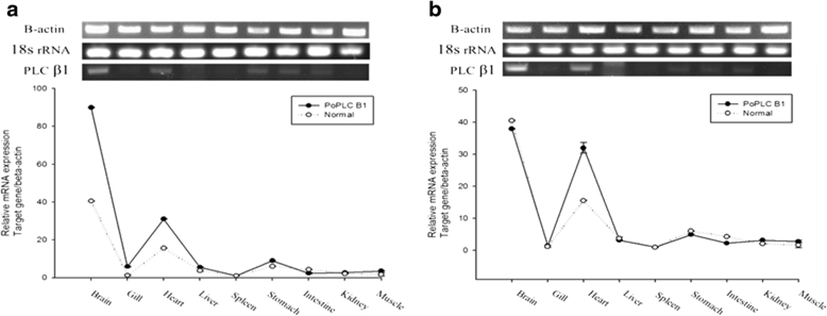
Discussion
In higher vertebrates, immunity can be broadly classified into adaptive immunity and innate immunity. Innate immunity is crucial for the recruitment of non-specific immune cells, including lymphocytes, dendritic cells, granulocytes, and macrophages, to the sites of infection by microorganisms and foreign substances. After these immune cells recognize the infection and kill the foreign cells, the adaptive immune system kicks in, which involves specific antigen presentation to B and T lymphocytes to fight the pathogen (Bonizzi and Karin 2004). Phospholipid second messengers produced by PLC-β have been shown to play a significant role in T lymphocyte chemotaxis (Smit et al. 2003). Furthermore, the PLC-β subfamily appears to be essential for the generation of IP3, DAG, and mobilization of Ca2+ (Bach et al. 2007). Moreover, PLC-β plays a critical role in activation of chemoattractant-induced integrin, cell-substrate adhesion, and in the movement and antibody production of B lymphocytes (Kawakami and Xiao 2013). Thus, PLC-βs are involved in the specialization and activation of immune cells, which regulate both the innate and adaptive immune responses (Forster et al. 1998). Previous studies have demonstrated that PLC-βs show tissue-specific expression patterns and are regulated by G protein. Indeed, in mammals, PLC-β1 is expressed in a wide range of tissues and cell types (Kawakami and Xiao 2013), including cardiac tissues (Jalili et al. 1999) and the brain (Suh et al. 2008).
PAMPs are characteristic molecular patterns that are highly conserved in different microorganisms (Savan and Sakai 2002). PAMPs, including LPS, ConA, and PolyI:C, activate immune system responses in the host. In particular, LPS is a constituent of the outer membrane of gram-negative bacteria and induces the proliferation of B lymphocytes (Estepa and Coll 1992). ConA is a plant lectin isolated from jack bean (Canavalia ensiformis) that was originally identified as a mitogen for T lymphocytes. It has been shown to activate lymphocyte proliferation by the production of lymphokines and monokines and to activate tumor necrosis factor-alpha, macrophages, and neutrophils (Xue and Bigio 2005). ConA also triggers a mechanism similar to that induced by the specific worm allergen (Panitch and McFarlin 1977). PolyI:C is a synthetic double-stranded RNA polymer that enhances the cytotoxicity of natural killer (NK) cells and macrophages (Biron 1997), increases interferon gamma (IFN-γ) production, and promotes lymphocyte adhesion to the endothelium (Doukas et al. 1994). Therefore, stimulation with LPS, ConA, and PolyI:C represent a challenge by bacteria, parasites, and viruses and were thus used to analyze the effects of immune challenge on PoPLC-β1.
There is significant evidence for cell-mediated responses after PolyI:C stimulation. NK cells respond to PolyI:C by releasing proinflammatory cytokines such as IL-6 and IL-8, as well as the antiviral cytokine IFN-α (Schmidt et al. 2004; Longhi et al. 2009). In addition, PolyI:C induces inflammation and long-lasting T cell immunity (Salem et al. 2006; Trumpfheller et al. 2008; Stahl-Hennig et al. 2009). In the present study, we observed a rapid reaction in the spleen and kidney, within 3 h following PolyI:C infection. The mRNA levels of IL-1β and IL-6 from the LPS, ConA, and PolyI:C infected organs were substantially upregulated and reached a peak at 3 or 6 h post injection. Our results indicated that PLC-β1 activation by pathogens induces inflammation cytokines including IL-1β and IL-6 production in the spleen and kidney tissues. Proinflammatory cytokines such as IL-1β and IL-6 can modulate the PLC-β and PIP2 signaling pathway (Zini et al. 2003). PLC-β activation pathway may possess the ability to modulate proinflammatory responses leading to downstream of NF-kB and interaction with G protein subunits (Townsend and Emala 2013). Also, PLC-β has been known as a central mediator of chemotaxis and plays a critical role in the recruitment of leukocyte to inflammatory tissues (Lehmann et al. 2008). Therefore, these results shed insight into the role of PLC-β1 in immune responses against pathogenic organism and provide a molecular foundation for further research, and monitoring in various teleost infection and immunity systems.
Stress factors in fish can be divided into two main categories: physical factors and chemical factors (Beckmann et al. 1990). The physical factors include salinity, temperature, density, and dissolved oxygen. In particular, salinity and temperature changes affect growth, reproduction, metabolism, osmoregulation, and immune function (Ackerman et al. 2000). Stress induced by changes in salinity has been associated with increased formation of ROS, which may considerably influence immune function and lead to oxidative stress (Paital and Chainy 2010; Shin et al. 2010). Furthermore, rapid changes in water temperature have been shown to enhance oxidative stress by increasing the amount of ROS generation in fish (Halliwell and Gutteridge 1999; Liu et al. 2007). Moreover, cells increase their antioxidant defense levels and associated enzymes in response to drastic temperature and salinity changes (Martinez-Alvarez et al. 2005). We observed increases in PoPLC-β1 in response to temperature and salinity stress in the brain and heart. There is evidence that the PLC-β1 level distinctly increases in response to ROS accumulation, which may play a role in protection against the damage induced by the physiological changes accompanying the stress response. This pattern of PLC-β1 expression may explain the process of protection from damage in these physiological responses (Yasuda et al. 2008). Since PLC-β1 can directly activate the PKCα and PKCβII isozymes, it is possible that overexpression of PLC-β1 could protect cells from oxidant-induced cell death via the activation of PKC (Lee et al. 2000).
Conclusions
In conclusion, our results showed that the PLC-β1 expression level increases in response to external stimuli mimicking immune challenge and stress in P. olivaceus. Research on the function of PLC-β1 and mechanisms controlling its expression in teleosts is limited, and our results provide clear evidence that the olive flounder PLC-β1 signal pathways may play a critical role in immune function at the cellular level and in inflammation reactions. In addition, PLC-β1 appears to act as an oxidative-stress suppressor to prevent cell damage in fish. Therefore, these results should serve as a foundation for further research on the piscine immune system and related signal pathways of lower vertebrates.
Abbreviations
ConA, concanavalin A; DAG, diacylglycerol; ER, endoplasmic reticulum; IP3, inositol 1,4,5-trisphosphate; LPS, lipopolysaccharide; PAMPs, pathogen-associated molecular pathogens; PH, pleckstrin homology; PIP2, phosphatidylinositol-4,5-bisphosphate PKC, protein kinase C; PLC, phosphoinositide-specific phospholipase C; PolyI:C, polyinosinic:polycytidylic acid; TIM, triose phosphate isomerase