Introduction
Homeostasis is a fundamental characteristic of life that resists perturbation and maintains stable system characteristics. For example, homeothermic organisms regulate a constant body temperature despite fluctuations in environmental temperatures (Eugster et al., 2008; Sterner, 2022). However, changes in the surrounding environment can disrupt homeostasis and impair biological functions. In ectothermic animals such as fish, environmental temperature changes trigger compensatory responses aimed at restoring balance and minimizing physiological disruptions caused by external stressors. To mitigate the effects of stressors on metabolism, fish exhibit a series of behavioral and physiological adaptations (Morris et al., 2013; Rossi et al., 2017).
Water temperature is one of the most influential factors affecting the growth and physiological performance of fish. Variations in water temperature can significantly impact normal metabolic activity and physiology, and when water temperatures exceed biological limits, physiological disorders, and homeostatic disturbances may lead to disease (Kumar et al., 2019). With rising water temperatures driven by global and local climate change, understanding how fish respond to thermal stress in terms of energy metabolism homeostasis has become increasingly important. Insights into these mechanisms are critical not only for advancing stress physiology research but also for developing practical solutions to promote aquaculture sustainability. To address stress from elevated water temperatures, several studies have explored alternatives, such as developing stress-resistant fish varieties, predicting physiological changes, or formulating feeds that enhance antioxidative capacity and immunity to cope with climate change (Lee et al., 2023). To ensure sustainable aquaculture in the face of climate change, it is crucial to establish a fundamental understanding of physiological responses, particularly energy metabolism homeostasis, under varying durations of thermal stress. Hematocrit and plasma metabolites, such as glucose (GLU) and cortisol, are sensitive indicators of stress and metabolic changes in fish, making them valuable parameters for analyzing the effects of acute temperature exposure (Wendelaar Bonga, 1997). However, there is currently a lack of information on how fish respond to different exposure durations of temperature stress in terms of energy metabolism homeostasis.
Olive flounder, a top-ranked aquaculture species in Korea, accounted for 46,000 tons of production in 2022, far surpassing other species (Statistics Korea; KOSTAT, 2023). Despite its economic importance, olive flounder faces sudden environmental changes associated with extreme climate events, such as elevated water temperatures during summer. This underscores the urgent need to investigate stress tolerance mechanisms in olive flounder, particularly under acute thermal exposure. In this study, the liver and brain were chosen as target organs due to their key roles in stress and metabolic regulation. The liver is central to energy metabolism, while the brain governs stress responses. These organs were prioritized to elucidate the mechanisms behind thermal stress adaptation in olive flounder. Therefore, the present study was conducted to evaluate the effects of acute temperature exposure duration on plasma metabolites and the relative gene expression levels involved in nutrient metabolism and heat shock responses in the liver and brain of juvenile olive flounder.
Materials and Methods
Juvenile olive flounder were obtained from the Garorim fish farm in Taean, Korea, and acclimated for 18 days (December 21, 2022–January 8, 2023) in 27 L tanks at Pukyong National University, Busan. During the acclimation period, the water temperature was maintained at 20.6 ± 0.09°C (mean ± SEM). The fish were fed a diet containing 20% fishmeal at 2.5% of their body weight per day and fasted for 24 hours prior to the experiment to minimize the effects of feeding on metabolism. At the start of the experiment, rectangular tanks were prepared with water temperatures of 30°C and 20°C to serve as the treatment and recovery groups, respectively. A total of 84 juveniles, with an average body weight of 25.0 ± 0.56 g (mean ± SEM), were randomly distributed into 21 rectangular tanks set to 30°C, with 4 fish per tank (n = 4 tanks per treatment). The fish were abruptly exposed to the high-water temperature (30°C) to simulate acute thermal stress conditions for varying durations of 2, 4, or 6 hours. Following acute temperature exposure, the experimental groups were transferred to recovery tanks maintained at 20°C. However, the 0-hour recovery group was sampled immediately after the high-temperature stress exposure. Samples of whole blood and tissues (liver and brain) were collected at 0, 2, 4, 6, 12, 24, and 48 hours post-exposure during the recovery phase (Fig. 1). This experimental setup enabled the assessment of both immediate and delayed physiological and molecular responses to acute temperature stress.
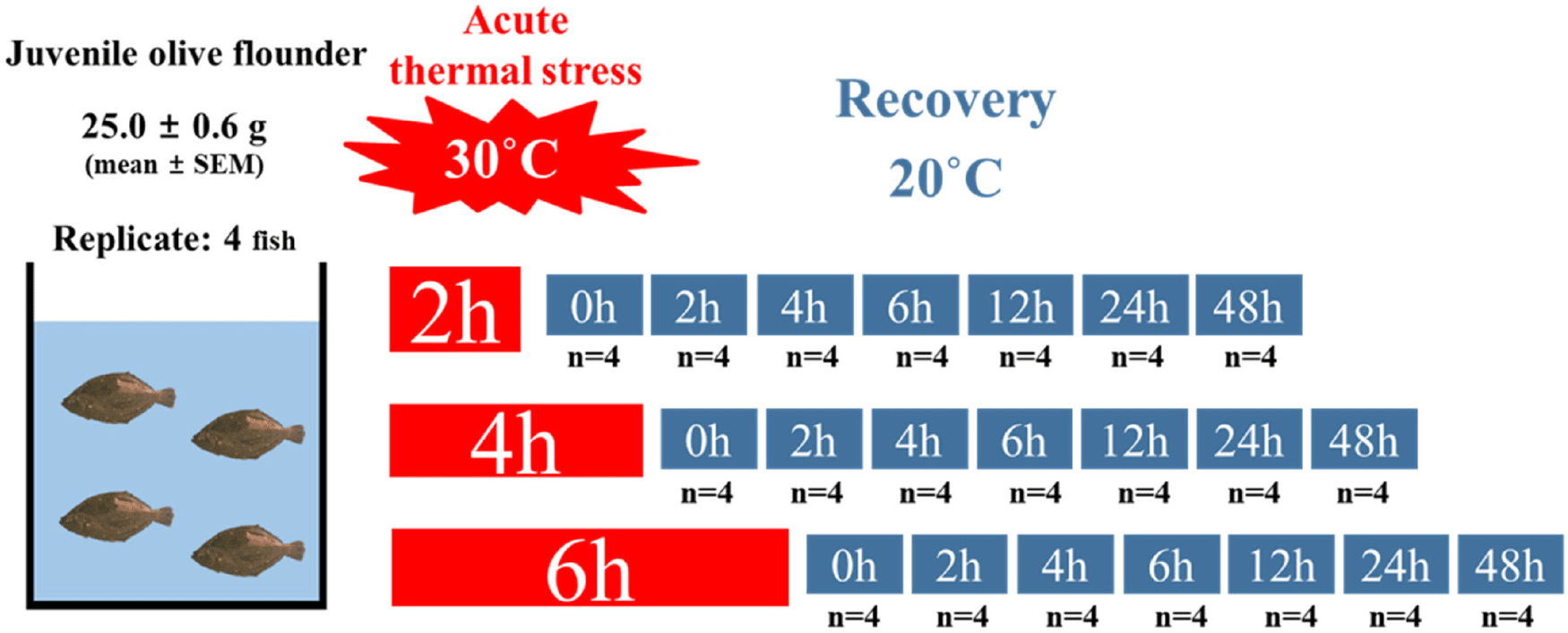
Blood, brain, and liver tissues were collected after the recovery period to analyze stress responses, immunity, and metabolic gene expression under acute heat stress. To minimize sampling stress, fish from each group were anesthetized using 300 ppm of 2-phenoxyethanol. Tissues were immediately frozen in liquid nitrogen and stored at −84°C until further analysis.
Total RNA was extracted following the manufacturer’s protocol for TRI-SolutionTM (TS200-001, Bio Science Technology, Seongnam, Korea). For each sample, 500 µL of TRI-Solution was used for homogenization with a bead mill homogenizer (OMNI Bead Ruptor Elite, Omni International, Kennesaw, GA, USA). After homogenization, 100 µL of chloroform (1/5th of the TRI-Solution volume) was added. The mixture was centrifuged at 9,660 × g for 15 minutes at 4°C, and the supernatant was transferred to a new tube. To precipitate RNA, 500 µL of isopropyl alcohol was added to the supernatant, and the mixture was centrifuged again at 9,660 × g for 10 minutes at 4°C. The resulting supernatant was discarded, and the RNA pellet was washed with 75% ethanol and air-dried completely. The dried RNA pellet was then dissolved in sterile distilled water and treated with DNase-I (Sigma-Aldrich, St. Louis, MO, USA) to remove genomic DNA contamination. The purity and quantity of the total RNA were evaluated using spectrophotometry (EonTM Microplate Spectrophotometer; BioTek, Winooski, VT, USA) by measuring absorbance ratios at A260/230 and A260/280. RNA samples were adjusted to a final concentration of 500 ng/µL and stored at –84°C until further use.
Blood samples were collected using a 1 mL syringe containing the anticoagulant dipotassium ethylenediaminetetraacetic acid. After blood extraction, hematocrit was analyzed immediately. The remaining blood was divided into microtubes and centrifuged at room temperature for 5 minutes at 11,000 ×g. The plasma was then stored at –84°C until further analysis. For plasma metabolite analysis, levels of glutamate oxaloacetate transaminase (GOT), glutamate pyruvic transaminase (GPT), total protein (TP), triglycerides (TG), total cholesterol (TCHO), and GLU were measured using Fuji Film DRI-CHEM SLIDE on a dry biochemical analyzer (Fuji DRI-CHEM NX500i, Fuji Photo Film, Tokyo, Japan). Cortisol concentrations were determined using enzyme-linked immunosorbent assay quantification kits (CSB-E08487f, CUSABIO, Houston, TX, USA) following the manufacturer’s protocol. After completing the procedure, optical density was measured at 450 nm using a microplate reader (AMR-100, Allsheng, Hangzhou, China) within 5 minutes.
The isolated RNA was converted into cDNA using an oligo (dT)18 primer and RT Premix (2X; SR-2000, Genetbio, Daejeon, Korea). Quantitative reverse-transcription polymerase chain reaction (qRT-PCR) was performed using the cDNA samples in Fast SYBR Green PCR Master Mix (Thermo Fisher Scientific, Waltham, MA, USA). The reaction was conducted under the following thermal cycling conditions: initial denaturation at 95°C for 20 seconds, annealing at 58°C for 30 seconds, and elongation at 60°C for 30 seconds. qRT-PCR analysis was performed for the following genes: heat shock protein 70 (hsp70), interferon regulatory factor 3 (irf3), glutathione peroxidase (gpx), cytochrome c oxidase (cox), adenosine-activated protein kinase beta (ampkβ), glucose-6-phosphatase (g6pase), peroxisome proliferator-activated receptor alpha (pparα), and peroxisome proliferator-activated receptors gamma (pparγ). The 18S rRNA gene was used as the internal reference. The reactions were run and analyzed using a QuantStudioTM 5 system (Applied Biosystems, Foster City, CA, USA). Relative mRNA expression levels were quantified using the 2^(-ΔΔCt) method. Primers for the experiment were designed using the Primer Express 3.0.1 program and validated for specificity through the NCBI database (Table 1). All qRT-PCR reactions were performed in triplicate.
PCR, polymerase chain reaction; hsp70, heat shock protein 70; irf3, interferon regulatory factor 3; gpx, glutathione peroxidase; ampkβ, adenosine-activated protein kinase beta; g6pase, glucose-6-phosphatase; pparα, peroxisome proliferator-activated receptor alpha; pparγ, peroxisome proliferator-activated receptors gamma.
The data were analyzed on an individual basis, with values from the same experimental groups averaged and expressed as mean ± SEM (n = 4). Statistical analysis was conducted using the R statistical software package (version 4.2.2). A one-way analysis of variance (ANOVA) was performed to assess the statistical significance of differences between group means. When significant differences were identified (p < 0.05), Tukey’s Honestly Significant Difference (HSD) test was used for post-hoc multiple comparisons.
Results
Tables 2, 3, and 4 present the hematocrit, plasma metabolites, and stress response in juvenile olive flounder acutely exposed to 30°C for 2, 4, and 6 hours, respectively. Hematocrit levels showed no statistically significant differences among the experimental groups (p > 0.05). However, plasma GOT and GPT levels increased significantly immediately after thermal exposure (p < 0.05) and gradually decreased during the recovery phase. TP, TG, and TCHO levels exhibited no significant differences across all exposure durations. In contrast, GLU and cortisol levels increased sharply immediately following heat stress exposure (p < 0.05) and decreased during the recovery phase.
Tables 5, 6, 7, 8, 9, and 10 present the relative gene expression levels in the liver and brain of juvenile olive flounder acutely exposed to 30°C for different durations. Expression levels of hsp70 were significantly affected by acute thermal stress, showing a sharp increase immediately after exposure, followed by a rapid decline during the recovery period in both the liver and brain (p < 0.05). The expression levels of irf3 and gpx tended to be significantly higher in the liver during the recovery period (p < 0.05). Conversely, cox, g6pase, ampkβ, pparα, and pparγ generally showed a decreasing trend during the recovery period as the exposure duration increased in both the liver and brain (p < 0.05), except for pparα in the liver after 6 hours of thermal stress exposure, which increased as recovery time progressed.
Values are means from triplicate groups of juvenile olive flounder where the values in each row with different superscripts are significantly different (mean ± SEM; p < 0.05).
Relative gene expression level (recovery time at 0 h was set as a control; 18s used as housekeeping gene).
hsp70, heat shock protein 70; irf3, interferon regulatory factor 3; gpx, glutathione peroxidase; cox, cytochrome c oxidase; ampkβ, adenosine-activated protein kinase beta; pparα, peroxisome proliferator-activated receptors alpha; pparγ, peroxisome proliferator-activated receptors gamma; g6pase, glucose-6-phosphatase.
Values are means from triplicate groups of juvenile olive flounder where the values in each row with different superscripts are significantly different (mean ± SEM; p < 0.05).
Relative gene expression level (recovery time at 0 h was set as a control; 18s used as housekeeping gene).
hsp70, heat shock protein 70; irf3, interferon regulatory factor 3; gpx, glutathione peroxidase; cox, cytochrome c oxidase; ampkβ, adenosine-activated protein kinase beta; pparα, peroxisome proliferator-activated receptors alpha; pparγ, peroxisome proliferator-activated receptors gamma; g6pase, glucose-6-phosphatase.
Values are means from triplicate groups of juvenile olive flounder where the values in each row with different superscripts are significantly different (mean ± SEM; p < 0.05).
Relative gene expression level (recovery time at 0 h was set as a control; 18s used as housekeeping gene).
hsp70, heat shock protein 70; irf3, interferon regulatory factor 3; gpx, glutathione peroxidase; cox, cytochrome c oxidase; ampkβ, adenosine-activated protein kinase beta; pparα, peroxisome proliferator-activated receptors alpha; pparγ, peroxisome proliferator-activated receptors gamma; g6pase, glucose-6-phosphatase.
Values are means from triplicate groups of juvenile olive flounder where the values in each row with different superscripts are significantly different (mean ± SEM; p < 0.05).
Relative gene expression level (recovery time at 0 h was set as a control; 18s used as housekeeping gene).
hsp70, heat shock protein 70; irf3, interferon regulatory factor 3; gpx, glutathione peroxidase; cox, cytochrome c oxidase; ampkβ, adenosine-activated protein kinase beta; pparα, peroxisome proliferator-activated receptors alpha; pparγ, peroxisome proliferator-activated receptors gamma; g6pase, glucose-6-phosphatase.
Values are means from triplicate groups of juvenile olive flounder where the values in each row with different superscripts are significantly different (mean ± SEM; p < 0.05).
Relative gene expression level (recovery time at 0 h was set as a control; 18s used as housekeeping gene).
hsp70, heat shock protein 70; irf3, interferon regulatory factor 3; gpx, glutathione peroxidase; cox, cytochrome c oxidase; ampkβ, adenosine-activated protein kinase beta; pparα, peroxisome proliferator-activated receptors alpha; pparγ, peroxisome proliferator-activated receptors gamma; g6pase, glucose-6-phosphatase.
Values are means from triplicate groups of juvenile olive flounder where the values in each row with different superscripts are significantly different (mean ± SEM; p < 0.05).
Relative gene expression level (recovery time at 0 h was set as a control; 18s used as housekeeping gene).
hsp70, heat shock protein 70; irf3, interferon regulatory factor 3; gpx, glutathione peroxidase; cox, cytochrome c oxidase; ampkβ, adenosine-activated protein kinase beta; pparα, peroxisome proliferator-activated receptors alpha; pparγ, peroxisome proliferator-activated receptors gamma; g6pase, glucose-6-phosphatase.
Discussion
Thermal stress is one of the most critical environmental challenges faced by aquatic animals. Acute thermal stress disrupts physiological and molecular homeostasis, leading to impaired growth, metabolic disorders, and increased mortality (Deng et al., 2020; Guillen et al., 2019; Logan & Somero, 2011; Pérez-Casanova et al., 2008). A comprehensive understanding of the physiological and genetic responses of fish to such stressors is essential for developing effective strategies to enhance aquaculture resilience (Volkoff & Rønnestad, 2020). The present study focuses on juvenile olive flounder, an economically important species in Korea, to investigate their physiological and molecular responses to acute temperature stress and subsequent recovery.
Hematocrit is known to be significantly affected by water temperature in fish and is considered a reliable indicator of physiological changes. For instance, a significant decrease in hematocrit was observed in Shortnose Sturgeon (Acipenser brevirostrum) following exposure to thermal stress at 31°C (Ziegeweid & Black, 2010). Similarly, decreased hematocrit levels were reported in juvenile sablefish (Anoplopoma fimbria) under above-optimal temperatures (Kim et al., 2019). In the present study, consistent with findings from previous research, hematocrit levels showed an initial increase immediately after exposure to acute thermal stress, followed by a gradual decrease during the recovery phase. This result suggests that acute thermal stress temporarily impacts hematocrit levels, likely as part of an immediate physiological adaptation to thermal stress, with recovery mechanisms subsequently facilitating the restoration of homeostasis over time.
In the present study, plasma metabolites, including GOT, GPT, GLU, and cortisol, were significantly affected by acute thermal stress. GOT and GPT, plasma-specific enzymes that respond sensitively to stress, were elevated immediately following thermal stress (Woo et al., 2018). Similarly, GLU and cortisol, widely recognized as stress markers in fish (Baeck et al., 2014; Fevolden et al., 2002), increased sharply after thermal stress and subsequently decreased during the recovery phase. Cortisol, a steroid hormone synthesized and released by interrenal tissue in the kidney, plays a critical role in regulating the stress response and blood GLU levels in fish (Jentoft et al., 2002; Lee et al., 2023; Wendelaar Bonga, 1997). Under stress conditions, cortisol stimulates gluconeogenesis to increase GLU production, providing energy to cope with stressors (Faught & Vijayan, 2016). In this study, the transient increases in GOT, GPT, GLU, and cortisol levels indicate that energy substrates were mobilized to maintain homeostasis during thermal stress and were subsequently restored during recovery. Interestingly, GPT levels showed no significant difference at 6 hours of exposure, which may suggest that recovery had already begun prior to sampling. This inconsistency underscores the importance of understanding how exposure duration influences metabolic responses, highlighting the need for further research. It is well known that thermal stress disrupts energy balance and alters lipid metabolism, including TCHO and TG (Liu et al., 2019). Similarly, studies have consistently reported the mobilization of energy substrates in stressed fish (Brandão et al., 2015). However, in this experiment, no significant differences or trends were observed in TP, TG, and TCHO levels, suggesting that homeostasis in these metabolites may not have been significantly affected.
In this experiment, gene expression analysis in the liver and brain revealed tissue-specific responses to acute thermal stress. hsp70, a molecular chaperone, exhibited significantly upregulated levels immediately after thermal stress, followed by a rapid decrease during recovery. This result aligns with previous findings that hsp70 is upregulated following acute thermal stress and normalizes during recovery (Lewis et al., 2010; Lund et al., 2003). gpx, an enzyme essential for antioxidant systems that mitigate oxidative damage caused by stress (Cheng et al., 2018; Srikanth et al., 2013), did not display a clear trend in expression levels in the liver and brain in this study. This suggests variability in oxidative stress responses depending on the duration of exposure and recovery time. Yuan et al. (2022) reported that irf3, a gene associated with innate immune function, was significantly affected by temperature stress in stone loach (Triplophysa bleekeri). In our study, significant differences in irf3 expression levels were observed in both the brain and liver, showing an upregulation trend during the recovery period. This may indicate the activation of stress recovery mechanisms, as both organs play vital roles in coordinating immune and metabolic responses. G6pase, a key enzyme in gluconeogenesis, showed elevated expression levels in the liver during the recovery period. However, no significant differences were observed at the 4-hour and 6-hour exposure intervals in the brain, emphasizing the liver’s primary role in energy metabolism and its importance in facilitating recovery after stress (Yuan et al., 2020). In contrast, ppars and cox did not show consistent trends, while ampkβ, a central regulator of cellular energy homeostasis (Bremer et al., 2016; Cantó & Auwerx, 2009), displayed increased expression in the liver in the 2-hour exposure group. However, in the 4-hour and 6-hour exposure groups, expression levels tended to decrease as the recovery period progressed. This suggests the dynamic involvement of ampkβ in maintaining energy balance during stress and recovery (Hardie et al., 2012).
In conclusion, this study demonstrated that acute thermal stress induces diverse physiological and molecular responses in juvenile olive flounder, highlighting the complexity of stress adaptation across tissues and metabolic pathways. The differential recovery rates observed in metabolites and gene expression levels indicate that thermal stress does not uniformly affect the fish but instead triggers tissue-specific and gene-specific adaptations. This research provides important insights into the mechanisms by which olive flounder cope with rapid environmental changes, such as those driven by climate change, offering foundational knowledge for enhancing aquaculture resilience. Additionally, plasma GLU, cortisol, and hsp70 expression were identified as potential markers for monitoring thermal stress and adaptation, providing practical tools for future research and aquaculture management. By identifying critical stress markers and their recovery dynamics, this study establishes a framework for improving olive flounder health and sustainability in aquaculture systems. Future research should focus on identifying stress markers under conditions of long-term chronic thermal stress or repeated thermal stress, particularly in relation to fish physiology and energy metabolism.