Introduction
Olive flounder (Paralichthys olivaceus) is a commercially valuable species in East Asian aquaculture, particularly along the southern coast of Korea. However, increasing sea surface temperatures and rising ocean heat content are significantly impacting marine ecosystems and aquaculture operations. In Korea waters, these changes have been accelerating at approximately 2.6 times the global average over the past 55 years (1968–2023; NIFS, 2023; Fig. 1). The prevalence of flow-through aquaculture systems, which rely on direct seawater intake, exposes olive flounder farms to these temperature fluctuations. This complicates the maintenance of optimal rearing conditions (Lee et al., 2023). As water temperatures rise due to climate change, fish in these systems increasingly face temperature stress which adversely affects their growth, immune function, and overall health.
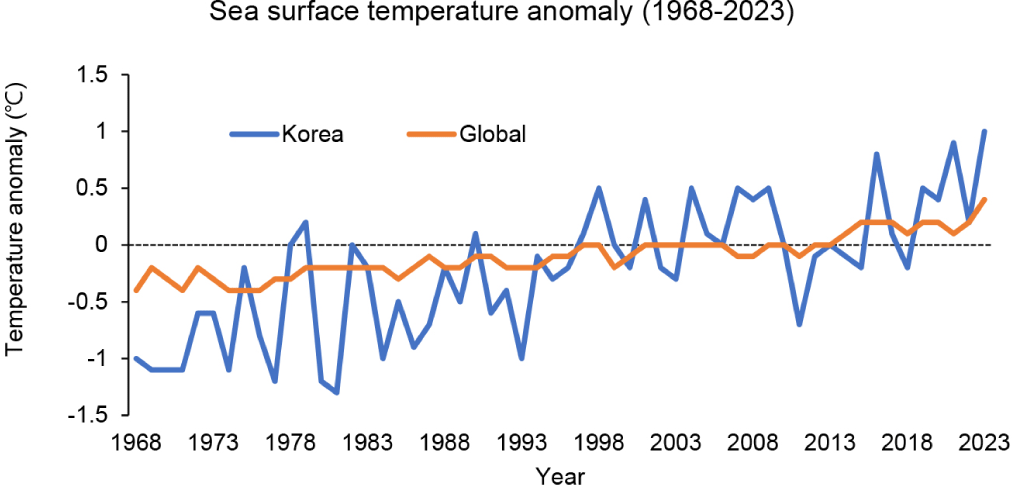
The optimal temperature range for the growth and metabolic activity of olive flounder is between 19.5°C and 25°C (Palaksha et al., 2008). Deviations from this optimal range, particularly acute temperature stress, can trigger both primary and secondary stress responses in fish. Primary stress responses include the activation of the hypothalamic-pituitary-interrenal axis, which leads to the release of stress hormones like cortisol (CORT). This hormonal cascade initiates secondary stress responses, involving alterations in plasma glucose (GLU) levels, electrolyte imbalances, and increased metabolic rates. These responses can ultimately compromise immune function, reduce growth, and increase mortality under chronic stress conditions (Ciji & Akhtar, 2021). Managing these physiological challenges has become a priority for sustainable aquaculture as climate-induced stressors become more frequent.
Taurine, also known as β-aminoethane sulphonic acid, is a naturally occurring β-amino acid widely distributed in various tissues throughout the body. Unlike other amino acids, taurine is not incorporated into proteins and primarily exists in its free form (O’Flaherty et al., 2003). The body synthesizes taurine mainly in the brain and liver from the sulfur-containing amino acids, such as methionine and cysteine via a reaction catalyzed by cysteine-sulphonic acid decarboxylase. Traditionally, taurine has been viewed as a mere end product of sulfur amino acid metabolism, with its primary physiological role being bile conjugation (O’Flaherty et al., 2003). However, recent research indicates that taurine possesses broader physiological functions, increasing interest in its potential benefits for fish health and stress tolerance across various species. For instance, studies on hybrid snakehead (Channa maculatus♀ × C. argus♂) indicated that taurine reduced ammonia stress by lowering blood urea nitrogen respiratory burst and blood cell apoptosis rate, relative mRNA expression of hepatic catalase (cat), and heat shock protein 70 (hsp70; Tan et al., 2018).
Nonetheless, the effects of taurine on growth and stress tolerance appear to be both species-specific and size-dependent. For instance, Kim et al. (2003) found that taurine supplementation was essential during the early growth stages of Japanese flounder (P. olivaceus), although its effects diminished in fingerlings. Similarly, in juvenile turbot, taurine improved growth performance at smaller sizes, while larger fish exhibited less pronounced benefits (Qi et al., 2012). These findings suggest that taurine’s efficacy may vary depending on the developmental stage of the fish and the specific environmental stressors they encounter.
Given the limited research on the physiological responses of olive flounder to acute temperature stress and the potential role of taurine in enhancing stress resilience, this study aimed to investigate the effects of taurine supplementation on the physiological and stress responses of juvenile olive flounder under elevated temperature conditions. We hypothesized that taurine supplementation would mitigate the negative effects of acute temperature stress, thereby improving survival, immune function, and antioxidant defense mechanisms. The results of this study will contribute to a deeper understanding of how dietary interventions can support aquaculture species facing climate-induced environmental challenges, ultimately aiding in the sustainability of aquaculture practices and food security.
Materials and Methods
The current study was reviewed and approved by the Institutional Animal Care and Use Committee (IACUC) of Pukyong National University, Busan, Korea (protocol code: 554). The principal investigator and research team were committed to ensuring the humane and ethical treatment of all animals involved in this study.
The detailed experimental design is illustrated in Fig. 2, and the ingredient composition of the basal diet is outlined in Table 1. Based on previous studies investigating taurine’s efficacy in aquaculture, we established varying concentrations to assess its potential effects on the physiological responses of juvenile olive flounder under acute temperature stress. Our experimental diet concentrations were strategically designed to encompass a range of taurine levels, allowing for a thorough evaluation of its impact on stress resilience. Seven isonitrogenous (approximately 54.5% crude protein), isolipidic (approximately 12.2% crude lipid), and isocaloric diets (approximately 5,049 kcal/kg gross energy) were prepared to include taurine at levels of 0%, 0.5%, 1%, 1.5%, 2%, 2.5%, and 3%. The analyzed taurine concentrations for each diet were as follows: 0.13% (named as Tau0.13), 0.56% (Tau0.56), 0.96% (Tau0.96), 1.5% (Tau1.50), 2.01% (Tau2.01), 2.54% (Tau2.54), and 2.87% (Tau2.87). The concentrations of taurine were verified using high-performance liquid chromatography (HPLC) to ensure accuracy and consistency across the dietary treatments. The diet formulation process began by mixing all the dry ingredients for 15 min using an electric mixer (HYVM-1214, Hanyoung Food Machinery, Hanam, Korea). Fish oil was then gradually added to the mixture, followed by an additional 15 min of mixing. Next, tap water, which constituted 45% of the total ingredient volume, was incorporated, and the mixture was thoroughly blended for another 15 min. The resulting wet dough was passed through a pelletizing machine (SFD-GT, Shinsung, Gwacheon, Korea) fitted with a flat die featuring holes approximately 2 mm in diameter. The pelleted strands were manually fragmented into smaller pieces and then oven-dried using a dryer (KE-010, Dongwon Industries, Seoul, Korea) at 45°C for 18 h. This process resulted in a moisture content below 10% after cooling. Once cooled to room temperature, the experimental diets were packaged, labeled according to their re-spective compositions, and securely stored in a freezer at –20°C until their scheduled use.
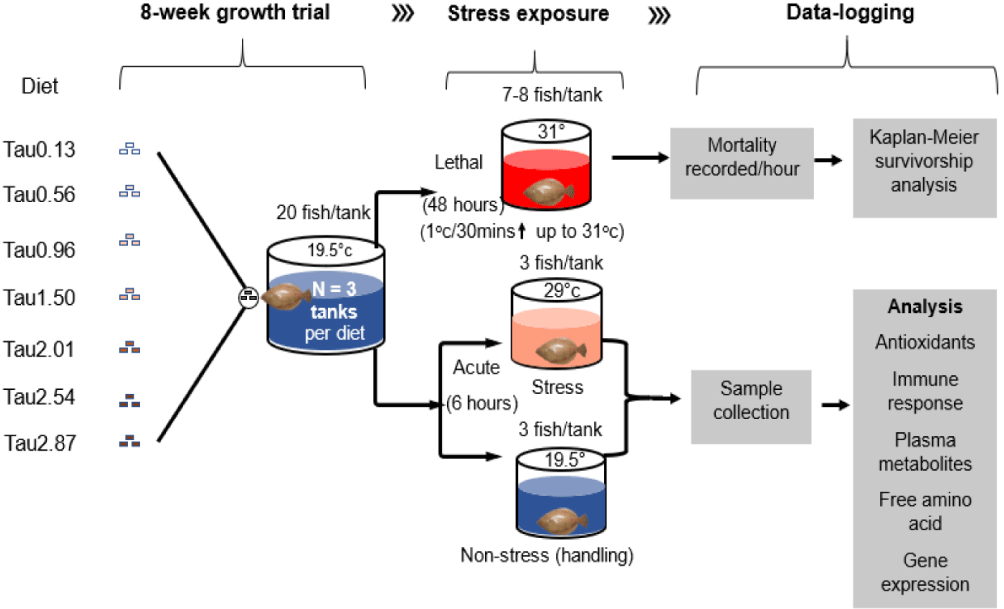
3) Mineral mix (as g/kg premix): Ferrous fumarate, 12.50; Manganese sulfate, 11.25; Dried ferrous sulfate, 20.0; Dried cupric sulfate, 1.25; Cobaltous sulfate, 0.75; Zinc sulfate KVP, 3.75; Calcium iodate, 0.75; Magnesium sulfate, 80.20; Aluminum hydroxide, 0.75.
The proximate composition of the experimental diets (Table 1) was determined using the methodology established by the Association of Official Analytical Chemists (AOAC, 2005). The moisture content was assessed by drying homogenized diet samples to a constant weight at 105°C. The ash content was quantified by incinerating samples at 550°C in a muffle furnace for 3 h. Nitrogen content (N × 6.25) was assessed through the kjeldahl method, which involves acid digestion using the 2300 Auto analyzer (Foss Tecator AB, Höganäs, Sweden). The crude lipid content was determined by ether extraction using the Soxtec System 1046 (Foss Tecator AB). The free amino acid profile of the diets was analyzed by the methodology described by Antoine et al. (1999).
The juveniles were sourced from a local fish farm (Samboo farm, Boryeong, Korea) and acclimated to the experimental conditions at the Aquafeed Nutrition Laboratory of the College of Fisheries, Pukyong National University, Busan. During the initial acclimation phase, they were fed a commercial diet (pellet size: 2mm; crud protein: > 52%, crude lipid: > 10%, crude ash: < 15%; Cargill Agri Purina, Seongnam, Korea) for one week. Following this period, they underwent a progressive transition to the diet (Tau0.13). The acclimation phase concluded when their feeding response reached a level of activity comparable to that observed during the initial stage with the commercial diet. The overall acclimation period lasted a total of three weeks.
Four hundred twenty juveniles with an initial weight averaging 12.97 ± 0.1 g (mean ± SEM) were randomly distributed into 21 (25-liter rectangular) tanks (20 fish per tank). The seven experimental diets were assigned randomly to the tanks in triplicate. An additional group of extra fish of the same size was kept in a separate tank of equal volume within the same rearing system and labeled ‘Extra’. This additional group was fed the Tau0.13 diet throughout the experimental period and was later used for a pre-exposure evaluation before the temperature stress exposure test.
Feeding occurred twice daily at 9:00 and 17:00 h over 8 weeks, with a fixed feeding rate of 2% to 3% body weight per day. Every other week, all fish in the tanks were weighed, and the feed amount was adjusted accordingly. After feeding, a 30-min interval was allowed before removing fecal materials. The tanks were connected to a semi-recirculatory system. This system included a protein skimmer (Model ORI-M, Dong yang engineering, Yangju, Korea), which removed organic compounds, proteins, and other impurities from the water. An electronic thermostat (DOV-887, Daeil, Busan, Korea) maintained a constant water temperature. This temperature was monitored every 10 min throughout the trial by a HOBO data logger (HOBO water temperature Pro v2 data logger-U22-001, Onset, Bourne, MA, USA). A flow rate of 2.5 L/min was maintained during the experimental period. The system was continuously replenished with fresh, UV-sterilized seawater after siphoning to compensate for water loss, and a complete water change was performed twice a week to maintain optimal conditions. The photoperiod remained consistent, providing 9 h of light followed by 12 h of darkness, facilitated by an artificial lighting system.
Daily water quality assessments included measurements of dissolved oxygen (DO) and pH using a Multi-Parameter Meter (YSI Model 58, YSI Incorporated, Yellow Springs, OH, USA). Nitrogenous wastes were monitored using a commercial kit, the API Saltwater Master Test Kit (Petco, San Diego, CA, USA). Water temperature was kept constant with an electronic thermostat (DOV-887, Daeil) and maintained at 19.5 ± 0.1°C. Throughout the experiment, DO levels were recorded at 7.98 ± 0.18 mg/L, pH at 7.38 ± 0.02, ammonia at 0.84 ± 0.06 mg/L, nitrite at 0.82 ± 0.11mg/L, and nitrate at 53.41 ± 2.34 mg/L.
At the end of the 8-week feeding trial, all fish (24-hour postprandial) in each tank were weighed to calculate growth performance indices, including weight gain (WG), specific growth rate (SGR), feed efficiency (FE), and survival rate (SR). The following equations were used for each index:
Three fish from each tank were euthanized with phenoxyethanol (500 ppm; Sigma Aldrich, St. Louis, MO, USA) and stored in a freezer (–20°C) until further analysis. The frozen fish were pooled, homogenized, and freeze-dried for whole-body proximate composition analysis through AOAC methodology (AOAC, 2005).
Before conducting the main lethal temperature exposure test, we performed a pre-exposure test to evaluate the suitability of the target temperature. Eight extra fish, which were kept on the Tau0.13 diet throughout the experimental period, were exposed to the same conditions planned for the main test. No mortality was observed during this pre-test before reaching the target temperature of 31°C, confirming that the chosen lethal temperature was appropriate for the main test. Subsequently, to investigate the whole-organism responses of the test diet-fed experimental fish over eight weeks, eight fish that were 24-hour postprandial were subjected to lethal temperature exposure. The temperature was incrementally raised at a rate of 1°C every 30 min, starting from the rearing temperature of 19.5°C and eventually reaching the target temperature of 31°C. This approach was similar to the procedure outlined by Lu et al. (2016). Fish survival was monitored and recorded hourly for a continuous 48-hour period, at which point the lethal stress experiment concluded. All tanks were equipped with aeration systems to ensure that DO levels remained near saturation. During the exposure test, a data logger (HOBO Water Temperature Pro v2 data logger-U22-001, Onset) was used to monitor the temperature throughout the experiment, recording data every 10 min.
A group of three test diet-fed juveniles (24-h postprandial) per tank was abruptly transferred into separate tanks filled with seawater at an elevated temperature of 29°C. This elevated temperature exposure lasted for six h. The selection of this temperature and the duration of exposure was based on the outcomes of a preliminary test conducted with the same group of juveniles. Importantly, this preliminary test revealed no observable mortality within 24 h post-exposure, indicating that the selected conditions did not result in ecological mortality and were therefore suitable for acclimation in our experiment. To investigate any stress effects (e.g., handling stress) apart from the temperature stress during the acute test, an additional set of three juveniles from each tank was moved from their original rearing tank to a separate tank maintained at the rearing temperature of 19.5°C. This handling stress exposure was sustained for six h, aligning with the duration of the acute stress exposure.
At the end of the exposure test, the fish were euthanized using 2-phenoxyethanol (500 ppm, Sigma Aldrich). Blood samples were collected from the caudal vein using a 1 mL syringe pre-treated with dipotassium ethylenediaminetetraacetic acid (EDTA; Bylabs, Hanam, Korea) as an anticoagulant. The collected blood samples from each tank were then pooled into a 3-mL siliconized vacuum tube treated with EDTA, divided into 1-mL microtubes, and immediately centrifuged at 11,000 rpm for 5 min. The resulting clear plasma was decanted into 1.5-mL microtubes and snap-frozen in liquid nitrogen until all samples had been collected. The plasma-filled microtubes were stored at –84°C until further analysis. Additionally, whole brain and liver samples were extracted from each individual from their respective tanks. These tissues were also snap-frozen in liquid nitrogen until all samples were collected and subsequently stored at –84°C until further analysis.
The free amino acid analysis of the samples after the growth trial was conducted using the method described by Antoine et al. (1999). A 0.3 g of tissue sample (whole brain and intestine) was homogenized using a hand-held homogenizer (Model D-130, Wiggens, Beijing, China). After homogenization, 10 mL of HPLC grade distilled water was added, and the mixture was vortexed for 1 min at 5,000 rpm using a high-performance multifunction vortex mixer (MaxshakeTM, Daihan Scientific, Wonju, Korea). This was followed by sonication for 20 min. The samples were then centrifuged at 4,500 rpm for 10 min at 4°C. From the resulting supernatant, 0.7 mL was placed into 1.5 mL microtubes, where 0.7 mL (at a ratio 1:1) of 7% sulfosalicylic acid was added. The mixture was kept in the dark at 4°C overnight. Afterward, it was centrifuged at 4,500 rpm for 10 min at 4°C, and a 1 mL of the supernatant was filled through a 0.2 micron filter and dispensed into HPLC vials for analysis. For plasma samples, the same steps were followed except for the homogenization step, which was skipped due to the liquid nature of the sample.
The plasma samples from both the stress group and non-stress group (handling) collected during the acute exposure test were analyzed for plasma metabolites, including GLU (#1050), total cholesterol (TCHO; #1450), triglycerides (TG; #1650), total protein (TP; #1850), glutamic oxaloacetic transaminase (GOT; #3150), and glutamic pyruvic transaminase (GPT; #3250). The analysis was conducted using a dry biochemical automatic analyzer (Fuji DRI-CHEM nx500i, Fuji Photo Film, Tokyo, Japan) following the manufacturer’s instruction.
The enzyme-linked immunosorbent assay (ELISA) quantification kits (Cusabio, Wuhan, China) were utilized according to the manufacturer’s instructions. A microplate reader (AMR-100, Allsheng, Hangzhou, China) set to an optical density of 450 nm facilitated the quantification process. The analyses performed included the assessment of antioxidant system indicators such as glutathione peroxidase (GPx; CSB-E15930Fh) and superoxide dismutase (SOD; CSB-E15929Fh). In addition, immunological parameters were evaluated, including lysozyme (LZM; CSB-E17296Fh) and immunoglobulin M (IgM; CSB-E12045Fh), along with CORT (CSB-E08487f) as a stress-related indicator in the plasma samples obtained after the acute exposure test.
The brain and liver tissues collected from the acute temperature exposure test underwent gene expression analysis. A sample of less than 100 mg was homogenized in a microtube containing 1-mL RiboExTM (GeneAll Biotechnology, Seoul, Korea) using a homogenizer (Model D-130, Wiggens). Total RNA was extracted using a commercial kit (Hybrid-RTM, GeneAll Biotechnology) following the manufacturer’s protocol. The concentration and purity of the RNA were checked with a NanoDrop ASP-2680 spectrophotometer (ACTgene, Piscataway, NJ, USA). It was confirmed that the purified RNA was free of DNA and proteins, with an A260/A280 ratio of 2.21 ± 0.01 (mean ± SEM). Complementary DNA (cDNA) was synthesized using a commercial kit (PrimeScriptTM 1st Strand cDNA Synthesis Kit, Takara Bio, Shiga, Japan) following the manufacturer’s protocol. The obtained cDNAs were used as templates for quantitative real-time polymerase chain reaction (PCR) to assess mRNA ex-pression levels of four selected stress-related genes, including heat shock protein 60 (hsp60), heat shock protein 70 (hsp70), heat shock protein 90 (hsp90), and the warm water stress-related gene (wap65). Beta-actin (β-actin) was used as the internal control. The primer sequences for the genes are listed in Table 2. The reaction was performed using the StepOne Real-Time PCR system (Applied Biosystems, Waltham, MA, USA) with TB® Green Premix Ex TaqTM II (Tli RNaseH Plus, Takara Bio). The qPCR program was set to 95°C for 30 s, followed by 40 cycles of 95°C for 5 s and 60°C for 30 s. After the reaction, a melting curve analysis was conducted to verify the specificity of the products. For each selected gene, qPCR reactions were performed on three replicate samples. The mRNA levels of the tested genes were normalized to the corresponding β-actin value and analyzed using the 2-△△Ct method (Liyak et al., 2001). Data analysis was performed using StepOne Software version 2.0 (Applied Biosystems).
Genes | Primer sequences | Tm (°C) | Accession number | Product size (bp) | Efficiency (%) | R 2 | Slope | Reference |
---|---|---|---|---|---|---|---|---|
β-actin | F: GGAATCCACGAGACCACCTACA | 62.1 | XM_020109620.1 | 264 | 99.9 | 0.9515 | 3.3250 | Nie et al. (2021) |
R: CTGCTTGCTGATCCACATCTGC | 62.1 | |||||||
hsp60 | F: TGACTTCGGGAAAGTCGGTG | 59.3 | XM_020105844.1 | 2927 | 100.1 | 0.8904 | 3.3200 | NCBI (2023) |
R: ACGATCTCCAGTGCACGTTT | 57.3 | |||||||
hsp70 | F: TTCAATGATTCTCAGAGGCAAGC | 58.9 | XM_020089177.1 | 113 | 99.4 | 0.9994 | 3.3364 | Mori et al. (2022) |
R: TTATCTAAGCCGTAGGCAATCGC | 60.6 | |||||||
hsp90 | F: GAGCGAGACAAGGAGGTGAG | 61.4 | XM_020091873.1 | 100 | 96.8 | 0.9944 | 3.4016 | Lee et al. (2023) |
R: CTGGCTTGTCTTCGTCCTTC | 59.3 | |||||||
wap65 | F: AACCAAGGCTGTGGGAAGAAAGAG | 63 | XM_020105098.1 | 1727 | 98.6 | 0.9398 | 3.3550 | Choi et al. (2010) |
R: GTGTCCGTGGAAGCAGTAGTAGTG | 64.4 |
Results of the growth response, whole-body proximate composition, and free amino acid profile obtained following the eight-week feeding trial were subjected to a one-way analysis of variance (ANOVA) using SAS version 9.4 (SAS Institute, Cary, NC, USA). For the survival analysis, we used R 4.4.1 (R Core Team, 2023) with the survival and survminer packages to perform Kaplan-Meier survival analysis. This approach allowed us to estimate survival curves and compare SRs among groups using the log-rank test. Specifically, the Kaplan-Meier method (via the survfit function) was employed to analyze survival data across different dietary treatments.
Results of the plasma metabolites, biomarker analyses, and relative gene expression levels obtained from the acute temperature exposure test were subjected to a two-way ANOVA to assess ttemperature exposure teste interactive effect between dietary treatment (different taurine levels in the diet) and temperature stress (non-stress exposure and stress exposure). Orthogonal polynomial regression analyses were performed in SAS version 9.4 (SAS Institute) using PROC REG to examine whether the measured responses to the graded taurine levels were linear or quadratic.
Regression analyes using one-slope straight broken-line, two-slope straight broken-line, quadratic broken-line, and quadratic models were conducted to determine the optimal inclusion levels of taurine based on the plasama free amino acids of juvenile olive flounder following the acute temperature stress exposure. These regression models were selected for their best fit to datasets encompassing dose-response relationships (Lee et al., 2014). Once estimates were obtained from each model analysis, model selection crtieria, including the Akaike information criterion corrected (AICc) and adjusted R-squared, were evaluted to select the best estimate among them. This regression analysis was conducted using the statitiscal software R 4.4.1 (R Core Team, 2023).
Before conducting ANOVA, data integrity was evaluated for assumptions, including normality and homogeneity of variance, using the Shapiro-Wilk and Levene’s tests, respectively. The results of these tests indicated that the assumptions of normality and homogeneity were met. Type III sums of squares ANOVA was employed to test all possible interactions or main effects. Significance was tested at p < 0.05. When significance was detected, a post-hoc test using Tukey’s HSD test for pairwise comparisons following ANOVA to evaluate differences between treatment groups. This test is specifically designed to control the family-wise error rate, reducing the risk of Type I errors that can arise from multiple comparisons.
Results
The effects of dietary taurine supplementation on the growth performance of juvenile olive flounder were assessed at the end of the eight-week experiment and are presented in Table 3 (top). No statistically significant differences (p > 0.05) were detected based on the ANOVA analysis for the measurments, including final body weight (FBW), WG, SGR, FE, SR, condition factor, hepatosomatic index, and viscerosomatic index (VSI). However, a significant quadratic trend was observed in FBW (p = 0.0262) and WG (p = 0.0099), indicating that these measurements increased with dietary taurine inclusion up to a maximum point before decreasing. Additionally, the lowest values for these measurements were found in the diet treatment containg the lowest taurine inclusion (Tau0.13).
The whole-body nutrient composition results of juvenile olive flounder fed the experimental diets for eight weeks are provided in Table 3 (bottom). Across all evaluated whole-body parameters, taurine supplementation at different inclusion levels did not lead to statistically significant variations, except for whole-body crude lipid levels. A significant reduction in crude lipid content was observed with increasing taurine supplementation compared to the Tau0.13 treatment, which was supported by a significant linear trend (p = 0.0090).
The cumulative SR of juvenile olive flounder exposed to lethal temperature stress for 48 h following the eight-week feeding trial is depicted in the Kaplan-Meier survivorship curve (Fig. 3). The first occurrence of mortality in all the diet groups occurred after the first eight h of exposure. However, at the end of the 48-hour exposure period, the SRs among all experimental groups were not significantly different.
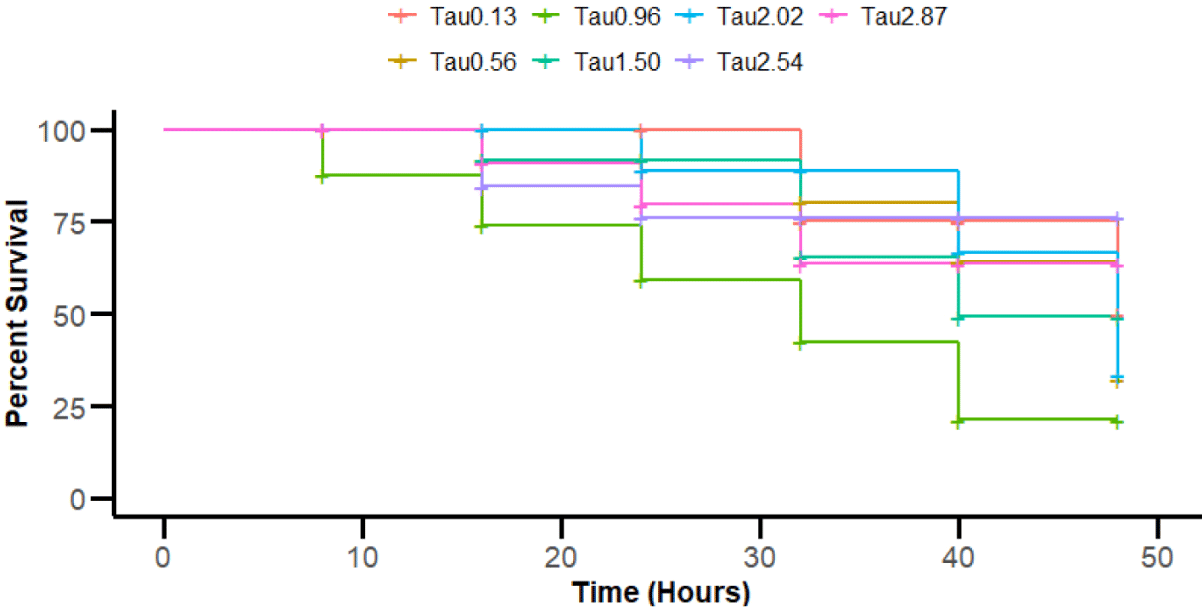
The free amino acid profile in the whole brain, intestine, and plasma of juvenile olive flounder fed the experimental diets for eight weeks was assessed. The results are presented in Tables 4 and 5, providing a comprehensive overview of the responses observed in each biological compartment.
Table 4 details the levels of free amino acids within the whole brain in response to varying levels of taurine supplementation. Taurine supplementation did not result in significant changes across all inclusion levels in the brain. In contrast, Table 5 shows that, while most amino acid exhibited no significant differences in the intestine, there was a significant accumulation of taurine across all tested levels except for the Tau1.50 diet. However, no dose-dependent accumulation pattern was observed in the taurine levels in response to dietary taurine inclusion.
On the other hand, in the plasma (Table 6), a more diverse response to taurine supplementation was observed, with several amino acids showing significant alterations. Phosphoserine, taurine, threonine, serine, glutamic acid, glycine, alanine, valine, cystine, methionine, leucine, tyrosine, phenylalanine, gamma-aminobutyric acid (GABA), and lysine all demonstrated significant changes across the taurine supplementation spectrum. The levels of these amino acids exhibited intricate patterns, with some displaying linear or quadratic trends in response to different levels of taurine supplementation. Therefore, regression analyses were conducted to determine the optimal inclusion levels of dietary taurine for the aforementioned free amino acids. Using regression models, inlcuding one-slope straight broken-line, two-slope straight broken-line, quadratic broken-line, and quadratic mdoels, the estimated optimal levels selected by the model selection criteria (AICc and adjusted R-squred) varied for each amino acid (Table 7). The lowest estimate was 0.64% for tyrosine, while the highest estimate was 2.25% for threonine.
1) The values represent the preferred values based on calculation of model selection criterion, including the Akaike information criterion corrected (AICc) and the adjusted R-squared values.
The interaction effect of temperature stress and taurine supplementaiton did not result in significant alterations in plasma levels of GOT, GPT, TG, TP, and TCHO (Table 8). However, a significant main effect of temperature stress on GOT and GPT levels was detected, showing that these tranaminases were dramatically elevated under temperature stress. Interestingly, a significant interactive effect between temperature stress and taurine supplementation on GLU levels was observed. While GLU levels did not change in response to varying dietary taurine levels, they were elevated when exposed to temperature stress. Notably, the treatment with the lowest taurine supplementation exhibited the highest GLU level compared to the other treatments under the stress exposure.
The results of GPx, SOD, IgM, LZM, and CORT in response to the interactive effects of temperature stress and dietary taurine supplementation are presented in Table 9. A significant main effect on IgM and LZM levels, as indices of immune response, was detected, indicating that their levels were highly reduced under stress. In contrast, the responses of GPx and SOD, which are antioxidant enzymes, were not comparable in response to both temperature stress and taurine supplementation. The GPx level was significantly reduced under stress but was not affected by taurine supplementation. Conversely, the SOD level was significantly elevated under temperature stress and was also influenced by taurine supplementation, with the lowest taurine level resulting in the highest SOD level.
Furthermore, a significant interactive effect between temperature stress and taurine supplementation on CORT levels were detected. No change in the CORT levels was observed under non-stress conditions; however, the levels were significantly affected by both temperature stress and taurine supplementation. This showed that the lowest taurine supplementation resulted in the highest CORT level under temperature stress.
The gene expression levels of stress-related parameters, including heat shock protein 60 (hsp60), heat shock protein 70 (hsp70), heat shock protein 90 (hsp90), and warm water stress-related gene (wap65), in the brain and liver of juvenile olive flounder fed the experimental diets and subjected to acute temperature stress are detailed in Tables 10 and 11. The results indicated no significant differences in the expression levels of these genes among the different dietary groups in both tissues.
Discussion
All experimental diets were readily accepted and rapidly consumed by the fish, minimizing the possibility of nutrient leaching into the water. In this study, we assessed the potential impact of taurine across various levels of organization in juvenile olive flounder. At the whole organism level, taurine supplementation did not significantly improve growth performance. Comparable findings have been reported in studies involving taurine supplementation in juvenile carp (Qi et al., 2012), and hybrid striped bass (Qi et al., 2012), where taurine supplementation did not also significantly enhance growth performance indices. However, Qi et al. (2012) found that in smaller turbot (6.3 g), feed intake and utilization improved with taurine supplementation, leading to significantly enhanced growth performance. Conversely, in larger turbot (165.9 g), taurine did not significantly enhance feed utilization. Similarly, Kim et al. (2003) reported that while taurine induced significant growth during the juvenile stage (initial size 0.4 g) of olive flounder, growth was not substantially affected in fingerlings (initial size 14.7 g). These findings suggest that the effects of taurine on growth may be both species-specific and size-specific, which could explain the lack of significant differences observed in our study with olive flounder juveniles averaging 13.0 ± 0.09 g at the beginning of the trial.
Additionally, our analysis of the whole-body proximate composition revealed no significant differences in moisture, crude protein, and crude ash content among the experimental groups, indicating that the dietary treatments did not affect these macronutrient levels in juvenile olive flounder. However, a significant reduction in crude lipid composition was observed, exhibiting a linear trend (p = 0.09) as the dietary taurine level increased. Similar results have been reported in studies involving the same species (Kim et al., 2008), Atlantic salmon (Espe et al., 2011), turbot (Qi et al., 2012), and red sea bream (Matsunari et al., 2008). The lower whole-body crude lipid content influences lipid metabolism and storage, affecting overall metabolism (Shi et al., 2022). Taurine plays a crucial role in fat digestion by conjugating bile acids, such as cholic acid and chenodeoxycholic acid, in the liver explaining the possible reason for the significant reduction in whole-body fat as observed in this study. In lipid metabolism, taurine is involved in the degradation of cholesterol metabolites and participates in micelle formation, which enhances fat absorption in the small intestine (Ferreira et al., 2014).
The deleterious effects of high temperatures are usually a consequence of energetic tradeoffs at organism levels with organisms tending to make higher energetic investments in physiological compensatory mechanisms, resulting in depletion of energy reserves, mainly lipids, especially in marine organisms where lipid provides the densest form of energy (Madeira et al., 2021). Therefore, future studies could investigate the mechanisms of action of taurine in lipid metabolism to gain additional insights into the possible role of taurine supplementation in managing lipid reserves in teleost fish during exposure to increased temperatures.
Furthermore, we investigated the potential effects of taurine supplementation on juvenile olive flounder at other levels of biological organization, analyzing free amino acids in the brain and intestine (organ level) and in the plasma (extracellular fluid). Taurine supplementation did not induce significant changes in free amino acid levels in the brain at any supplementation level, suggesting a robust regulatory mechanism, likely maintained by the blood-brain barrier, which controls the entry of amino acids into the central nervous system (Segarra et al., 2021). In contrast, the intestine exhibited significant taurine accumulation across most inclusion levels, except for the Tau1.50 group, where levels did not significantly differ from the Tau0.13 group. This finding implies effective dietary taurine absorption and highlights its potential role in digestive health (O’Flaherty et al., 2003). According to Wu (2020), taurine is absorbed intact by the intestinal mucosa, entering the portal circulation without degradation.
Regarding the lethal stress exposure of juvenile olive flounder after the feeding trial, there was no significant difference in cumulative SR after the 48-h exposure period among the various taurine supplementation groups. Although initial variations in survival were observed, particularly with the 0.13% and 1.50% taurine groups showing delayed mortality, these differences diminished over time. By the end of the trial, cumulative SRs across all taurine levels converged, suggesting that taurine supplementation did not provide a sustained long-term advantage in survival under our tested conditions. This indicates that the effect of taurine on juvenile olive flounder under extreme temperatures may be limited. The mechanism underlying taurine’s limited effect on olive flounder survival in extreme temperatures should be further investigated.
Nutrients dissolved in plasma can enter into the tissues from the capillaries through diffusion, filtration, and osmosis. In this study, our assessment of juvenile olive flounder’s plasma revealed significant alterations in several free amino acids, including branched-chain amino acids (valine, leucine, and isoleucine), taurine, phosphoserine, threonine, serine, glutamic acid, glycine, alanine, cystine, methionine, tyrosine, phenylalanine, and GABA. This suggests that taurine influences various metabolic pathways and interactions among amino acids, thereby providing a substrate pool for energy or protein synthesis (Hardy & Kaushik, 2021). As these amino acids may be redirected where metabolic demand is highest, sufficient availability of these free amino acids may support the organism’s adaptive response (Hardy & Kaushik, 2021; Fig. 4). Therefore, the elevated levels of free amino acids may indicate enhanced stress resilience and improved physiological responses in olive flounder. For instance, increased branched-chain amino acids suggest a heightened potential for protein synthesis and energy production, particularly under stress. Elevated plasma leucine supports muscle protein synthesis and metabolic regulation. Additionally, juveniles fed taurine-supplemented diets showed significantly higher plasma alanine, a key gluconeogenic amino acid, indicating improved energy regulation during metabolic stress. Elevated methionine, cysteine, and taurine levels may enhance antioxidant activity through glutathione synthesis (Métayer et al., 2008), further promoting stress resilience. The rise in plasma tyrosine and phenylalanine levels suggests an increased potential for neurotransmitter synthesis, potentially improving stress responses via catecholamine modulation, such as dopamine and norepinephrine (Fernstrom & Fernstrom, 2007). Additionally, the significant increase in GABA levels may further indicate taurine’s role in supplying GABA to regulate neural excitability, potentially offering neuroprotective effects during stress (Mezzomo et al., 2017). Overall, the observed changes in plasma amino acid levels suggest that taurine supplementation may play a crucial role in enhancing stress resilience and metabolic plasticity across various physiological pathways, particularly when the availability of key nutrients is limited due to environmental stress.
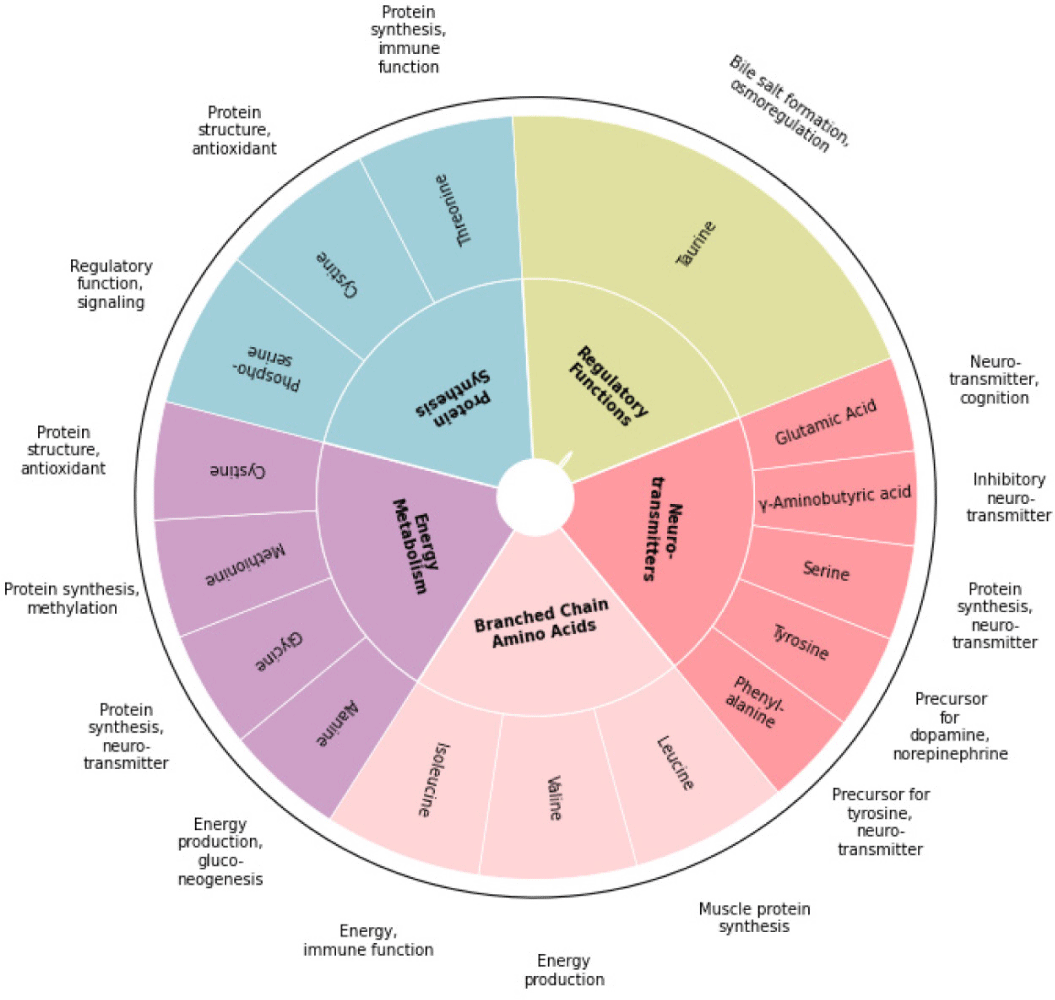
Regarding the assessment of plasma metabolites, particularly those related to liver function, GLU metabolism, lipid metabolism, and protein status, we observed that temperature is a key factor modulating some of these metabolites. Elevated temperatures significantly increased levels of GOT, GPT, and GLU at 29°C compared to 19.5°C. GOT and GPT, common indicators of liver health, were markedly higher at the elevated temperature, suggesting stress and potential liver damage. Similar observations were made in Nile tilapia (Oreochromis niloticus; Hegazi et al., 2010) under environmental stress. The rise in plasma GLU supports the notion of a stress response, as hyperglycemia is often associated with physiological stress. However, despite the significant effects of temperature on liver function and GLU metabolism, no significant changes were observed in plasma TG, TCHO, or TP levels. This finding aligns with those of Han et al. (2014), who reported that taurine supplementation did not significantly alter blood parameters such as hematocrit, hemoglobin, GLU, TP, TCHO, total bilirubin, and TG in olive flounder. Our results suggest that while temperature stress primarily disrupts liver function and GLU regulation, olive flounder may have mechanisms to maintain lipid and protein metabolism under temperature stress. This could be due to the short duration of temperature exposure or adaptive responses that buffer lipid and protein homeostasis during short-term thermal challenges. A key aspect of such adaptation in ectotherms is homeoviscous adaptation, where membrane lipid composition is adjusted to restore fluidity during temperature fluctuations by altering glycerophospholipid ratios. These mechanisms may contribute to the stability of lipid and protein metabolism in olive flounder under temperature stress (Ernst et al., 2016).
Additionally, we observed a significant interaction between taurine supplementation and temperature for GLU levels with higher taurine diets showing reduced GLU concentrations at elevated temperatures, emphasizing taurine’s role in alleviating stress-induced metabolic disturbances, particularly in GLU regulation. The reduction in GLU concentrations with increased taurine supplementation, especially at higher temperatures, suggests that taurine may play a protective role against stress-induced hyperglycemia (Kaplan et al., 2004). This finding is consistent with those reported by Qian et al. (2021) in hybrid grouper (♀ Epinephelus fuscoguttatus × ♂ E. lanceolatus) fed taurine-supplemented diets.
Furthermore, we investigated the effect of taurine on antioxidant enzyme activities, immune responses, and stress hormone levels of juvenile olive flounder after stress exposure. Our results revealed that temperature, rather than taurine supplementation, had the most significant impact on these parameters. Levels of GPx, IgM, and LZM levels all significantly decreased at higher temperatures, while levels of SOD and CORT were significantly increased. Additionally, we observed a significant interaction between temperature and diet concerning CORT levels. The Tau0.94 group exhibited a reduction compared to the control group at 29°C. This suggests that taurine supplementation may mitigate the stress-induced rise in CORT. This finding aligns with studies showing that taurine supplementation reduces stress hormone levels in juvenile rock bream (O. fasciatus; Ferreira et al., 2014).
While taurine’s primary role in stress mitigation is often linked to its antioxidant and osmoregulatory functions, its effects on the immune system are also noteworthy. Research on red sea bream (Matsunari et al., 2008) and Atlantic salmon (Espe et al., 2011) has shown that taurine can enhance immune function by modulating antioxidant enzyme activity. However, the absence of significant interaction effects on SOD, GPx, IgM, and LZM in our study suggests that taurine alone may not be sufficient to counteract the physiological impacts of acute temperature stress on these parameters in juvenile olive flounder.
At the molecular level, gene expression analysis of stress-related parameters in the brain and liver of juvenile olive flounder subjected to acute temperature stress showed no significant differences in the mRNA levels of key stress-related genes (hsp60, hsp70, hsp90, and wap65) among the different dietary groups. This indicates that taurine supplementation may not significantly influence the expression of these genes under acute temperature stress conditions. Given the critical roles these genes play in cellular stress management, other mechanisms, such as post-translational modifications or metabolic pathways, may be more directly involved in the physiological response to temperature stress.
Interestingly, several studies have reported that taurine supplementation enhanced the expression of stress-related genes in other fish species, such as hybrid snakehead (C. maculatus♀ × C. argus♂) and yellow catfish (Pelteobagrus fulvidraco) under ammonia stress (Tan et al., 2018). However, in species like Siberian sturgeon (Acipenser baerii) and rainbow trout, the expression of the hsp70 gene was not affected (Shi et al., 2020). This variation suggests that taurine’s effect on stress-related gene expression may depend on the type of stressor and the species involved.
Conclusion
The results of our study provide valuable insights into the response of juvenile olive flounder to taurine supplementation across various levels of biological organization. While taurine did not significantly enhance growth performance, it demonstrated a notable metabolic influence, particularly by reducing whole-body crude lipid content. This reduction aligns with taurine’s established role in lipid metabolism, which facilitates fat digestion and absorption through bile acid conjugation and micelle formation. Furthermore, taurine supplementation significantly altered the plasma free amino acid profile when exposed to the acute temperature stress. These alterations suggest that taurine has the potential to contribute to energy regulation and metabolic adaptation by providing a reservoir of amino acids that can be utilized during periods of increased metabolic demand.
At the molecular level, our findings indicate that taurine may play a role in stress regulation by helping to mitigate the elevation of CORT levels during acute temperature stress. This points to a potential stress-attenuating role of taurine, which may help fish cope with environmental challenges. However, the expression levels of key stress-related genes (hsp60, hsp70, hsp90, and wap65) remained unchanged across dietary groups, indicating that taurine’s protective effects may not involve direct modulation of these genes under acute temperature stress conditions. Instead, other mechanisms such as post-translational modifications or metabolic pathways may be more influential.
While taurine did not significantly affect immune responses or antioxidant enzyme activity, it underscores the complexity of its role in fish physiology. In conclusion, although taurine supplementation did not improve growth in juvenile olive flounder, its influence on fat metabolism, plasma free amino acid profiles, and stress hormone regulation suggests potential metabolic and stress-related benefits under specific conditions. Further research is needed to optimize taurine inclusion levels and investigate its long-term effects, particularly in environments subject to temperature fluctuations, with the goal of enhancing the resilience and overall health of aquaculture species.