Introduction
Houndfish (Tylosurus crocodilus) is a seafood containing good nutritional value with all essential amino acids for human health. However, microorganisms and autolytic enzyme activities cause quality deterioration, such as changes in the flavor and texture of the fish (Ibrahim et al., 2011). Traditionally, preservatives have been added to preserve and prolong the shelf-life of seafood products. However, adding preservatives raises concerns about food safety due to chemical hazards affecting consumers’ health. To eliminate the usage of preservatives, food packaging is employed to protect food from chemical contamination and foodborne pathogens that are harmful to consumers (Flórez et al., 2022). Recently, petroleum-based films such as plastic have been extensively used in food packaging due to their properties and economic aspects. However, petroleum materials are well known for their negative environmental and human health impacts (Khan et al., 2021). Thus, bio-based coating with biodegradability and non-toxicity has gradually replaced plastic materials in food packaging (Flórez et al., 2022). The edible coating can reduce moisture loss and maintain the flavor and texture of the food. In addition, the food product and coating materials can be consumed together, reducing environmental contamination (Ibrahim et al., 2011).
Chitosan is a natural biopolymer widely studied for food packaging applications in recent decades (Flórez et al., 2022). Regarding structure, chitosan is a partially deacetylated product of chitin under alkaline treatment (Bakshi et al., 2020). The molecular weight of commercial chitosan typically ranges from 50–2,000 kDa, with deacetylation degrees ranging from 50%–100% (Mourya et al., 2011). Chitosan can be grouped into three groups i.e., high molecular weight (HMW; > 1,000 kDa), medium molecular weight (MMW; 100–1,000 kDa), and low molecular weight (< 100 kDa) based on molecular weight (Gonçalves et al., 2021).
Moreover, chitosan displayed structural similarity to cellulose, with hydroxyl groups at carbon 2 replaced by acetamido or amino groups (Ibrahim et al., 2011). Chitin and chitosan contain 6.29% more nitrogen than most polysaccharides, which contain only carbon, hydrogen, and oxygen (Flórez et al., 2022). Chitosan is a promising biopolymer for film or coatings due to its remarkable oxygen barrier and film-forming abilities. In addition, chitosan and its derivatives are famous for biodegradability, non-toxic, antimicrobial activities, and extendibility, making them a potential candidate for the food packaging industry (Flórez et al., 2022).
Previous studies have demonstrated the effectiveness of chitosan coating on fish fillets; however, its utility for on-skin fish has not been extensively studied. In addition, houndfish have been found in the ocean worldwide (Froese & Pauly, 2024), and captured houndfish might deteriorate when not appropriately preserved. This study aimed to investigate the applicability of MMW chitosan for on-skin houndfish (T. crocodilus) coating under 20-day ice storage conditions.
Materials and Methods
Fresh houndfish (T. crocodilus) was captured from Nam-Du, Kien-Giang, Vietnam. The chitosan source (produced from Penaeus vannamei shrimp) was manufactured by Nha Trang University with Mw of 185 ± 15 kDa–MMW chitosan; the degree of deacetylation of 88.3 ± 2.2%, apparent viscosity of 169 ± 5.25 cPs, degree of solubility > 99%, mineral content of 0.82 ± 0.05%, and crude protein content of 0.67 ± 0.07%. All chemicals in this study were of analytical grade, e.g., boric acid, thiobarbituric acid (TBA), malondialdehyde dichloromethane, magnesium oxide, and Folin-Ciocalt were provided by Sigma-Aldrich. Nutrient broth (NB), Tryptic soy broth (TSB), and plate count agar (PCA) were obtained from Merck.
After pretreatment, gutted houndfish without head was iced at a ratio of 1:1 (w:w) in insulated polystyrene boxes and shipped to the laboratory within 4 hours. Fish (weight from 400 g to 600 g) were randomly divided into six groups (36 fish per group). Six different solutions were used for coating houndfish. The two treatments were uncoated controls consisting of distilled water (DW) and a 1% acetic acid solution (1% AA) in DW. The others were a 0.5% chitosan in 1% v/v AA (chitosan [CTS] 1), a 1% w/v chitosan in 1% v/v AA (CTS 2), a 1.5% w/v chitosan in 1% v/v AA (CTS 3), a 2% w/v chitosan in 1% v/v AA (CTS 4) (Fan et al., 2009). The chitosan solutions were continuously stirred to obtain the complete dispersion of chitosan before coating. The apparent viscosity and pH values of CTS 1, CTS 2, CTS 3, CTS 4 were 54.37 ± 1.15, 169 ± 5.25, 505 ± 12.1, 1,829 ± 143.5 cPs, and 2.87 ± 0.06, 3.25 ± 0.03, 3.46 ± 0.03, 3.54 ± 0.04, respectively. The pH of 1% AA was 2.4 ± 0.02.
Each houndfish was coated with MMW chitosan solutions at different concentrations according to the dipping method (1:1, w/v) for a fixed time of 2 min. Two control fish samples were soaked in 1% AA solution and DW for 2 min. After dipping, the fish-treated chitosan solutions were coated, removed, drained, and hung vertically. The chitosan coating layer was formed uniformly by air drying for 30 min in a cool room (< 20°C). This experiment recorded that the higher the chitosan concentration, the more viscosity. Thus, by the same dipping and drying time with other treatments, the chitosan coating with a 2% concentration applied to the fish was too viscous, resulting in some of the coating solution being lost and a reduction in layer thickness. After that, the fish was packaged in PA bags and stored with ice in polystyrene boxes (1 layer of fish and one layer of ice with the top layer and the last layer being ice to ensure the storage temperature was always < 4°C). All treatments were randomly sampled and analyzed on days 0, 4, 8, 12, 16, 18, and 20. Fish was debonded and deskinned to take flesh meat for sampling and analysis.
Two bacterial species were tested for the antibacterial activity of chitosan, including the Gram-negative Vibrio cholerae and the Gram-positive Staphylococcus aureus. The antibacterial activity test was carried out according to the method of Minh et al. (2019) with slight modifications as follows: chitosan solutions (2% w/v) were prepared in 1% AA solution in DW. Calculated amounts of these solutions were added to the liquid TSB medium (TSB, Merck, Darmstadt, Germany) to give a final MMW chitosan concentration of 0.5%, 1%, 1.5%, and 2%. After inoculation with 105 CFU of either bacterial strain, the medium was shaken at 50 rpm at 37°C for 24 h. After that, the culture was subcultured in a specific medium (NB medium for S. aureus, TSB medium for V. cholerae) and incubated at 37°C for 12 h to check bacteria growth. The number of colonies was counted and multiplied by the dilution constant to obtain the number of bacteria. The antibacterial effect (%) of the chitosan solutions (0.5%, 1%, 1.5%, 2 wt%) and 1% AA solution was determined using Equation (1).
where control is the number of bacteria in tap water (used for fish washing), and test sample is the number of bacteria in MMW chitosan solutions with different concentrations and 1% AA solution.
The fish mince (15 g) was mixed with 15 mL of 0.15 M KCl and homogenized. pH values were determined using a pH meter (Mettler Toledo, Columbus, OH, USA) according to Hultmann et al. (2012).
To determine WHC of minced fish, the centrifugation method described by Ofstad et al. (1993) was followed. 1.5 g of minced fish was placed in a 15 mL centrifugal tube and then centrifuged at 300 g for 10 min at 4°C using a Mikro 22-R centrifuge (Hettich zentrifugen, Tuttlingen, Germany). WHC is given as a fraction of water bound after centrifugation (% of total water).
M1 is the mass of empty centrifuge tube (g). M2 and M3 are the masses of the centrifuge tubes containing the sample before and after centrifuging, respectively (g).
Fish flesh was analyzed for hardness (maximum compression force) using a TA-XT2i Texture Analyzer (Stable Micro Systems, Godalming, UK). The double compression test (texture profile analysis) was performed using a 75 mm diameter cylinder probe (P75) (Saavedra et al., 2017). The highest thickness of fish flesh with cubes of 30 × 30 mm was kept at 4°C before being compressed at 50% of the original height, with a pretest speed of 1.0 mm/s, a test speed of 2.0 mm/s, and a posttest speed (5.0 mm/s). Texture analysis was performed at room temperature (20 ± 2°C).
Five grams of sample were placed in a Kjeldahl tube following the method described by Velho (2001). After that, 2 g of MgO and 50 mL of deionized water were added to the tube. Distillation was carried out for 5 min in a flask containing 25 mL of 1% boric acid and an indicator mixture of methyl red and bromocresol green (35 mg: 50 mg); then, the boric acid solution was titrated with a 0.1 N sulfuric acid solution.
Fish samples (25 g) were taken aseptically and diluted to a decimal dilution. PCA medium was mixed with the diluted sample and added to a sterile petri dish. The dish was incubated at 30°C for 48 hours, and the number of viable aerobic microorganisms per gram of sample was determined from the number of colonies counted on a selected plate. TVC was determined using the pour plate method, NMKL No. 86 (NMKL, 2013).
Thiobarbituric acid reactive substances (TBARs) were measured using the spectral colorimetric method developed by Raharjo et al. (1992). The sample was ground and then extracted with 5% TCA. After centrifugation at 1,050×g for 15 min at 4°C, the supernatant was collected and brought up to a volume of 50.0 mL. In test tubes, 2 mL of sample solution, 2 mL of 1,1,3,3-tetraethoxypropane (TEP) solution, and 2 mL of 80 mM TBA were added, respectively. The solution was stirred for 15 seconds and then placed in a water bath at 94°C for 5 min. The sample was cooled, and the absorbance was measured at 530 nm. Calibration curves were created using increasing TEP concentrations ranging from 0–10 μM/L. The TBARs were expressed as mg malonaldehyde (MDA)/kg sample.
The TCA-soluble peptide content of each sample was determined using the method developed by Benjakul et al. (2002). A sample weighing 3 g was homogenized with 27 mL of cold 5% TCA solution at a speed of 11,000 rpm for one min. The resulting homogenate was then kept in ice for 30 min and centrifuged at 5,000×g for 20 min at 4°C. The soluble peptides in the supernatant were measured using the Lowry method (Lowry et al., 1951) and expressed in µmol tyrosine per gram of muscle.
Sodium dodecyl sulfate-polyacrylamide gel electrophoresis (SDS-PAGE) was conducted using Laemmli’s (1970) method with minor modifications. A muscle sample (0.5 g) was dissolved in 20 mL of SDS-urea solution (20 mM Tris-HCl, pH 8.8; 2% SDS; 8 M urea; 2% β-mercaptoethanol) and stirred overnight. Electrophoresis was performed on a 10% running gel and a 4% stacking gel at 20 mA per gel using Blotter Set-SDS Page (Bio-Rad, Criterion cell, Hercules, CA, USA). Ten µg of protein was loaded into one lane of polyacrylamide gel. After separation, proteins were fixed and stained using methanol/acetic acid/water (30:10:60, v/v/v) containing 0.1% coomassie brilliant blue R-250. To obtain the final gel image, the gel was destained with a decolorizing solution (methanol:acetic acid:DW = 30:10:60, v/v/v).
Color parameters, including lightness (L*), redness (a*), and yellowness (b*), were measured on the surface of the flesh side of the fillets using a colorimeter. The instrument used for this purpose was PCE-CSM 2, manufactured by PCE Instruments in Meschede, Germany, or Beijing, China. The total color change (ΔE), which indicates the overall difference in color between the tested sample and the original sample at day 0, was calculated using the following equation.
The subscript 0 denotes the initial colour value of the fillet, and t denotes the colour value of the fillet on the day of the test.
The sensory properties of houndfish were measured by six trained panelists following the method described by Rong et al. (2009). The fillets for each treatment, measuring 3.0 cm in width and 2.0 cm in length, were prepared, wrapped in aluminum foil, and labeled with three-digit codes. Afterward, the samples were cooked for 10 min at 85°C using a water bath. Finally, the cooked samples were served on a white paper plate under fluorescent white light conditions. Samples were evaluated based on appearance, taste, odor, and texture using a 9-point scale. The scale values represent the panelists’ reactions as follows: 1, extreme dislike; 2, very much dislike; 3, moderate dislike; 4, slight dislike; 5, neutral; 6, like slightly; 7, like moderately; 8, like very much; 9, like significantly. The sensory evaluation resulted in a score of four, considered the threshold for determining acceptability.
Results
The antimicrobial activities of chitosan on S. aureus and V. cholerae are shown in Table 1 and Fig. 1. Chitosan showed antibacterial activities on both gram-positive (S. aureus) and gram-negative (V. cholerae). The antibacterial effect of 2% chitosan was 86.85% for S. aureus and 85.17% for V. cholerae. Meanwhile, AA showed antibacterial effects on S. aureus and V. cholerae, which were 38.70% and 37.45%, respectively. The antibacterial effect was positively correlated with the concentration of chitosan (0.5%–2%). In addition, significant differences in antibacterial effect between chitosan and control (i.e., 1% acetic acid) treatments were observed (p < 0.05).
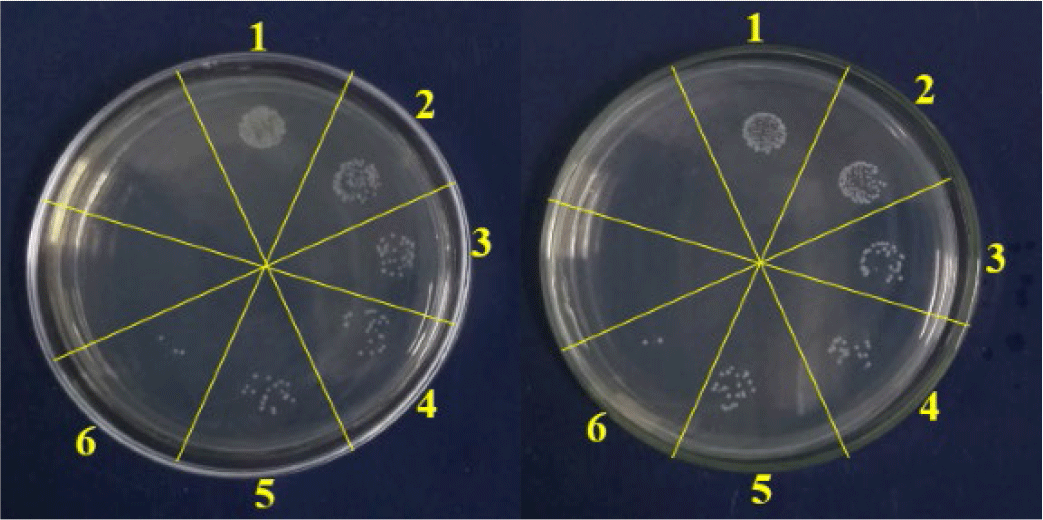
The pH changes are shown in Table 2. In general, the initial pHs were from 6.09 to 6.17 for all fish samples and increased to values of 6.3–6.51 as time progressed. The pH values were significantly higher in the DW treatment than in chitosan-coated samples from day 8 onwards (p < 0.05). The pH of samples coated with 1.5% chitosan remained consistently lower and more stable than those coated with 1% and 0.5% chitosan during the storage process (p < 0.05). However, samples coated with 2% chitosan did not exhibit a significant difference in pH maintenance compared to those coated with 0.5% and 1% chitosan from day 0–16 and throughout the storage period, respectively. However, there was no significant difference in pH values between samples coated in 1.5% and 2% chitosan.
WHC is the percentage of bound water retained in the mince after centrifugation. The higher the percentage of bound water retained in the mince, the more water leakage out of fish. The results showed that the initial WHC in the 82.1%–83.2% range increased gradually to 86.1%–92.3% as the experiment progressed (Table 3). There was no significant difference in WHC values in the first four days of the experiment. However, from day 8 to day 20, there was a significant difference in WHC values between the DW and chitosan treatments (p < 0.05). In addition, from day 16 to day 20, WHC values were significantly lower in 1%–2% chitosan-coated samples than in the two controls treated with DW and 1% AA (p < 0.05).
The hardness changes in houndfish are shown in Fig. 2. The hardness values decreased gradually as time progressed. There was no significant difference in hardness values between treatments on the first 12 days of the experiment. However, the hardness from the two controls was lower than that of 1.5% and 2% chitosan-coated samples from day 16 to day 20 (p < 0.05).
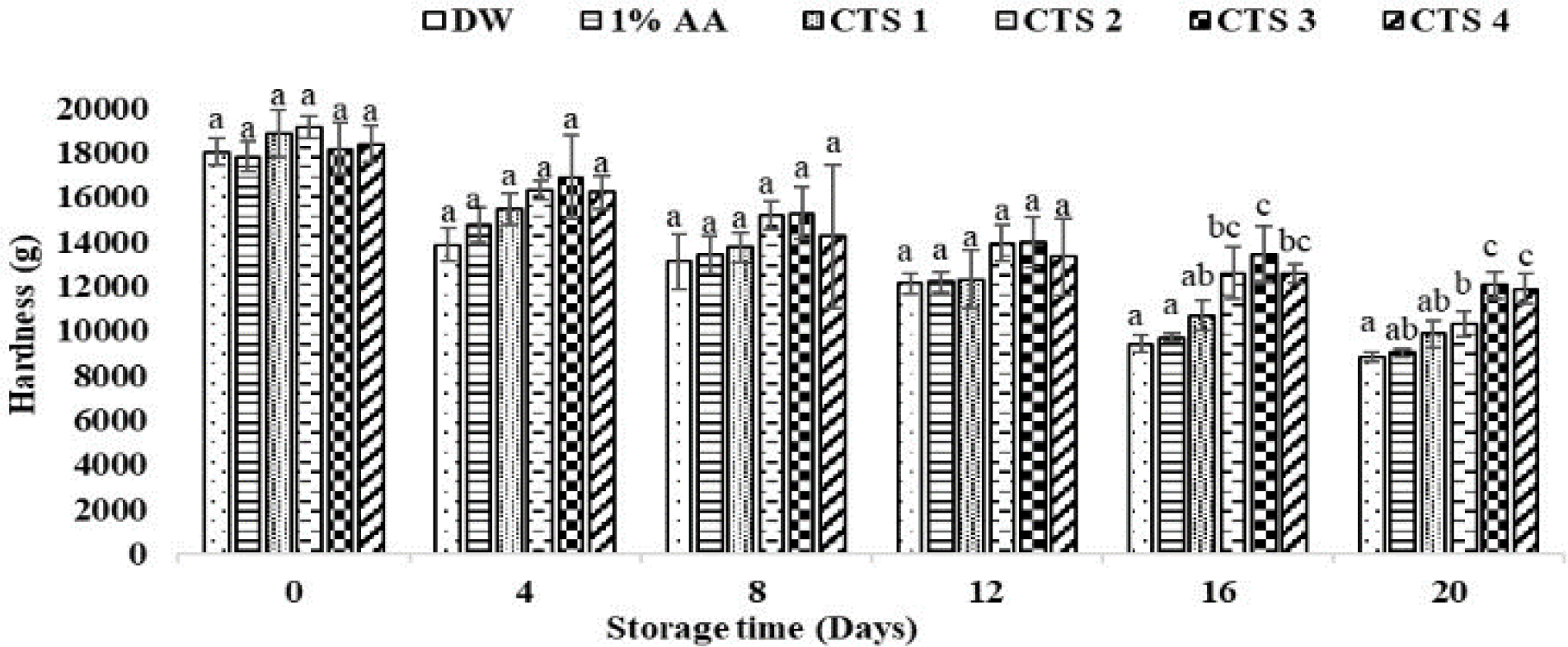
The TVB-N values of ice-stored houndfish are shown in Table 4. Initially, TVB-N values were not significantly different among all treatments, ranging from 8.33–8.53 mg N/100 g. There were significant differences (p < 0.05) in TVB-N values between DW and chitosan-treated samples from day 4 to day 20. At the end of the experiment, the TVB-N values from 1.5% and 2% chitosan-coated samples were 16.3 and 16.7 mg N/100 g, which were significantly lower than those in DW (22.2 mg N/100 g), 1% AA (20.3 mg N/100 g), and 0.5% chitosan treatments (18.5 mg N/100 g).
Lipid oxidation is a chemical process that leads to the development of rancidity, discoloration, and off-flavors in food. The TBAR values increased in the first 12 days and then decreased at the last storage day (Table 5). Generally, there was no noticeable difference in TBARs between chitosan concentrations, but from day 8, 1.5% and 2% chitosan treatments were significantly lower than the two control treatments. On day 20, the TBAR values from the control groups were 0.71 and 0.55 mg MDA/kg, which were significantly higher than chitosan-treated groups (1%, 1.5%, and 2%), with TBARs ranging from 0.45 to 0.47 (mg MDA/kg) (p < 0.05).
The TCA-soluble peptides can indicate the degree of protein degradation. As shown in Table 6, the TCA-soluble peptide concentration of samples elevated continuously from 0.22 to 2.96 µmol tyrosine/g during the entire storage period. The TCA-soluble peptide concentration from all treatments increased sharply in the first four days and the last eight days of the experiment. The TCA-soluble peptide content of fish coated with 1.5% and 2% chitosan was the lowest, while the highest was shown in the two control samples at the last period (p < 0.05). On day 20, the peptide concentration in the DW group was 2.04-fold and 1.75-fold higher than in the 1.5% and 2% chitosan-treated groups, respectively. Significant differences between the DW group and chitosan-coated groups were shown from day 16, while compared to fish coated with 1.5% and 2% chitosan, it was illustrated from day 8.
SDS-PAGE patterns of myofibrillar proteins of houndfish with treatments during ice storage at days 0, 4, and 18 are shown in Fig. 3. Characteristic bands of myosin heavy chain (MHC), actin, tropomyosin, troponin, and α-actinin are present in the gel. Myosin and actin are the major proteins that contribute to most of the functional properties of myofibrillar proteins. The appearance of band A from day 4 was noticed for all treatments, while the increasing intensity of bands I, II, III, IV, and V was observed with increasing storage time. The intensity of bands I of control samples (DW and 1% AA) increased on day 4 compared to day 1 but decreased on day 18. On day 18, the 1.5% and 2% chitosan treatments showed less intensity of band V, but significantly higher intensity of bands A, III, and IV than the two controls.
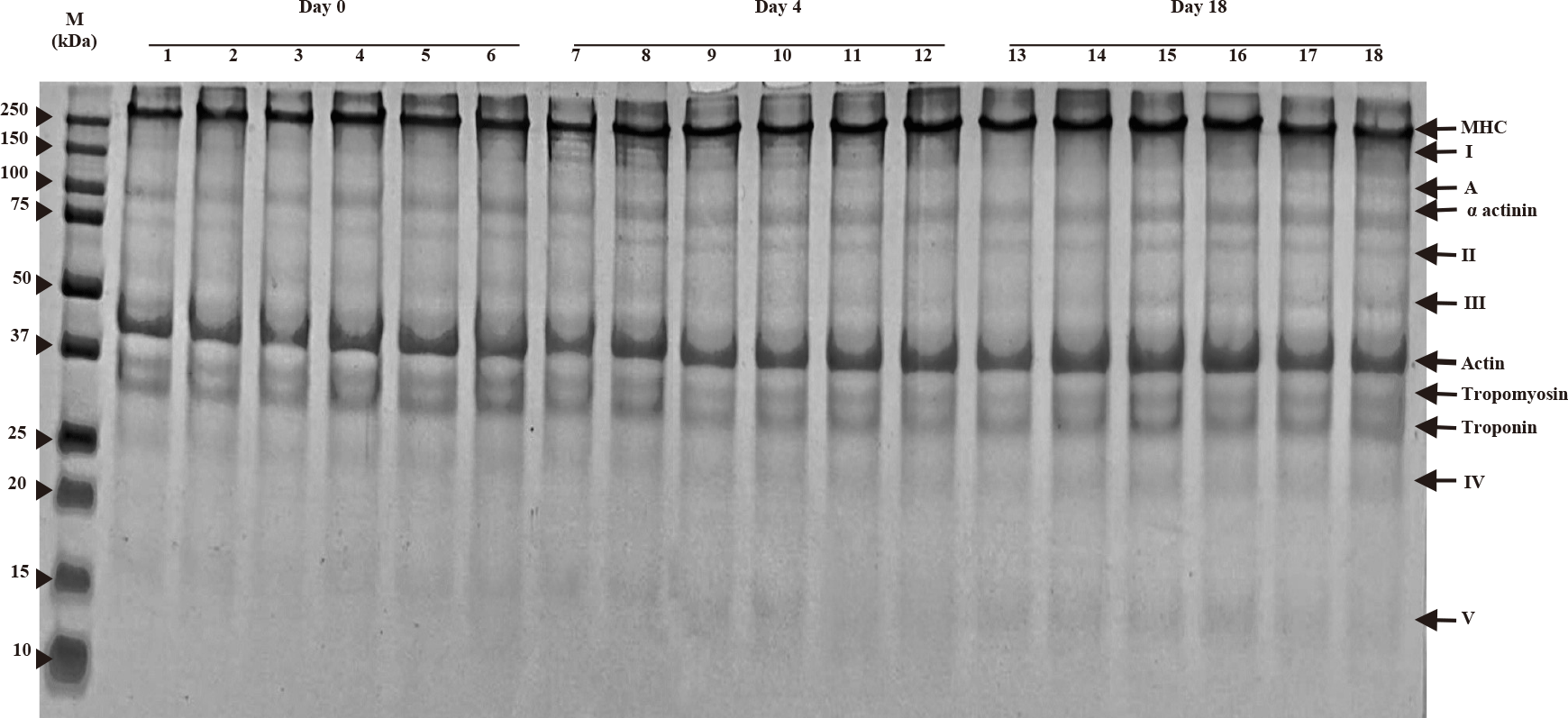
TVC in treatments on the first day in the range of 2.18–2.3 increased to 5.35–6.71 Log10 CFU/g after 20 days of storage, as shown in Table 7. The TVC values of the samples did not have a statistically significant difference on day 0. Starting from day 4, TVC had differences among treatments. In particular, the TVC of the two control samples was significantly higher than those treated with chitosan at different concentrations. The TVC of the two control samples started to increase strongly after eight days of cold storage, while that of the samples with chitosan coating increased strongly after 16 days. TVC of both controls exceeded 6 Log10 CFU/g on day 20, but not in the remaining samples. On the last day of storage, the 0.5% chitosan treatment had a significantly higher TVC than the 1.5% and 2% chitosan treatments.
As can be seen in Table 8, the color lightness (L* value) of fish muscle in all treatments on the first day was in the range of 49.5–52.4, decreasing gradually over 20 storage days to reach 42.7–47.0. The significant differences in lightness among treatments began to show from day 12 onwards. Specifically, chitosan-coated fish had the highest L* value, while that of fish treated with DW was the lowest (p < 0.05). Although fish muscle treated with 1% AA showed better lightness than fish treated with DW on day 12 (p < 0.05), differences between these two treatments were not observed on the remaining days. The most remarkable change of the total color change (ΔE) at each sampling day was observed for the DW treatment, followed by the 1% AA treatment (p < 0.05), while the treatment with (1%–2%) chitosan coating showed the least color change during storage.
The sensory scores on day 0 ranged from 8.50 to 8.93 for all treatment groups and gradually decreased with storage time (Fig. 4). From day 12 to day 20 of storage, the two control groups and 0.5% chitosan coating group had lower sensory scores that were significantly different from the coated sample groups with 1%, 1.5%, and 2% chitosan (p < 0.05). On days 16th and 20th, fish meat treated with DW and 1% AA turned slightly yellowish and had a sickly taste, fishy smell, and soft texture, leading to the lowest sensory scores of 3.40 vs. 3.93 and 2.8 vs 3.1 (< 4), respectively. In contrast, the 1.5% and 2% chitosan treatments had the highest sensory scores of 6.57 and 6.6 at the end of storage.
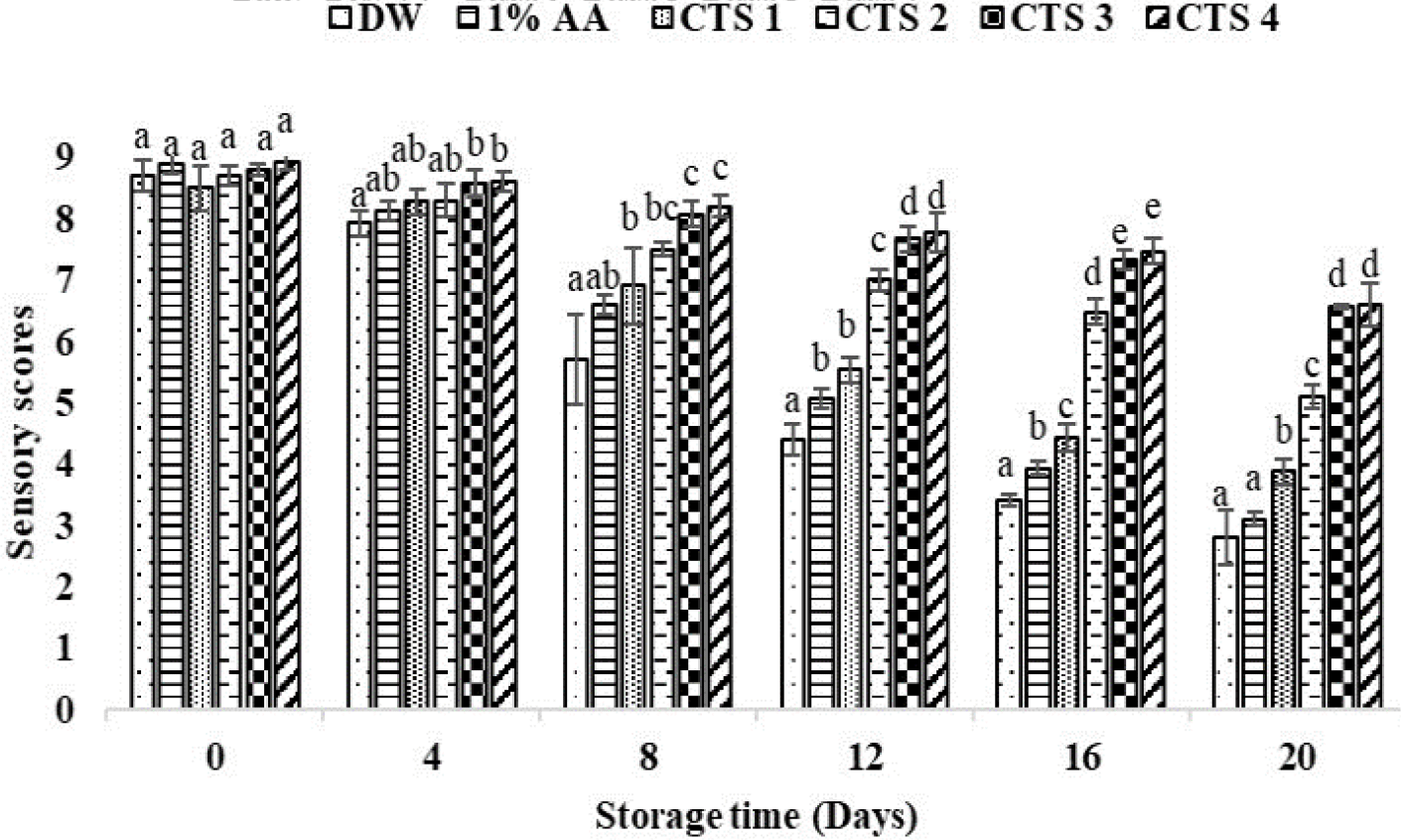
Discussion
Regarding the antimicrobial activity of chitosan, Priyadarshi & Rhim (2020) reviewed several mechanisms (1) chitosan’s cationic group interacts with the anionic bacterial cell wall peptidoglycans, dissolving the cell wall and causing cell death; (2) chitosan forms a barrier around the bacterial cell, preventing the exchange of solutes. This results in the failure of metabolic processes and cell death; (3) low MW chitosan can penetrate the cell nucleus and inhibit DNA translation by binding to DNA; (4) the quaternized chitosan can chelate essential trace metals, reducing their availability to microbes, inhibiting their metabolism, and causing their death.
In this study, MMW chitosan showed the antibacterial effect of chitosan on both gram-positive and gram-negative bacteria (S. aureus and V. cholerae). Moreover, the increased concentration of chitosan slowed bacterial growth, consistent with the study of Lagat et al. (2021), who suggested that increasing chitosan concentration contributed to improving the bacterial inhibition ability of chitosan.
On the first day of preservation, the pH values of houndfish were consistent with the study of Grigorakis et al. (2003) showing that the pH of the sample depends on the season and species. During storage, the pH value of the treatments showed an upward trend even though the fluctuations were also observed. One possible explanation implied that there may be the formation of basic decomposition products, such as ammonia and trimethylamine produced by spoilage bacteria and endogenous enzymes (Yang et al., 2019) in fish muscle through the postmortem period. The pH values of the sample treated by DW were higher than those of 1% acid acetic and chitosan due to the acid in the coating solutions. The 1.5% sample had a pH that remained lower and more stable than the 1% and 0.5 % samples, which was statistically attributed to the chitosan effect at higher concentrations. The 2% sample did not show this difference even though the 2% sample had fish meat pH no different from the 1.5% sample. This can be attributed to the less effective properties of the coating layer created from 2% chitosan solution (described above in preparation of coated houndfish). The lower pH of the chitosan-coated samples might enhance microbial inhibition and possibly inhibit the activity of the endogenous proteases. This result is consistent with the study on cold preservation of tilapia fillets with chitosan by Yang et al. (2019). This shows that the MMW chitosan coating not only maintains the pH value of the fillets but also on gutted fish with skin.
The WHC of food products affects their economic value and quality. At the end of storage, houndfish coated at 1%–2% chitosan had significantly lower WHC than the uncoated samples. Sathivel (2005) reported that chitosan coating was effective in reducing about 50% moisture loss of pink salmon fillets compared to the control uncoated ones. The chitosan coating likely reduced the shrinkage of the myofibrils, which in turn minimized water leakage from the fish muscle.
The textural property, particularly the hardness of all treatments in this study, decreased throughout the iced-stored period. Protein denaturation during storage can weaken connective tissue in fish muscle, leading to a reduction in texture hardness and quality loss (Piedrahíta Márquez et al., 2018). The texture softening could be induced by proteolysis resulting from the differential role of endogenous enzymes (e.g., cathepsins) and microorganisms (Ge et al., 2016). In this regard, the textural results of MMW chitosan-coated houndfish were consistent with the WHC results. Among treatments, the coated houndfish at 1%–2% chitosan could maintain better hardness during iced storage. These results were consistent with chitosan-coated Channa argus (Yang et al., 2014). According to Xu et al. (2014), the ability to control proteolytic enzyme activities in muscle tissue was responsible for the rapid destruction of cells and structural changes post-mortem. Bacterial enzymes are also involved in the later stages of degradation (Yang et al., 2014).
During cold storage, the TVB-N content of the samples increased due to microbial and enzymatic activity that decomposes proteins, resulting in the formation of trimethylamine, dimethylamine, and ammonia. These compounds, along with other volatile compounds, contribute to the increase in TVB-N content (Ruiz-Capillas & Moral, 2005). After 20 days of ice storage, all treatments involved uncoated houndfish had TVB-N that did not reach the rejection limit. Bacterial catabolism of amino acids in fish muscle caused an accumulation of ammonia and volatile bases (Fraser & Sumar, 1998). In this experiment, the coatings of 1.5% and 2% chitosan showed lower TVB-N values, indicating a significant adverse effect on bacterial growth (to be discussed later). This observed result was substantiated by other studies in terms of a decrease in TVB-N production by chitosan antimicrobial activity (Yang et al., 2019).
The recommended limit for TBARS value in fresh fish consumption is 5 mg MDA/kg (Sallam, 2007). In this study, MMW chitosan concentrations of 1.5% and 2% might decelerate the lipid oxidation in houndfish flesh based on lower TBARs than the remaining controls. A decrease in TBARs of all samples from day 12–20 was also found due to the direct microbial utilization of malonaldehyde and other TBARs or to reactions between these TBARs and the amine compounds induced by bacterial metabolism (Rhee et al., 1997). In addition, applying chitosan coating on seafood has been found to reduce lipid oxidation, e.g., tilapia (Yang et al., 2019).
Chitosan’s mechanism as an antioxidant against lipid oxidation is due to the formation of stable compounds between amino groups and volatile aldehydes, such as malondialdehyde (Souza et al., 2010). Another antioxidant effect is to chelate ion metals and/or bind to lipids (Xue et al., 1998). The chitosan coating layer on the product surface also acts as a barrier to oxygen permeability, preventing oxygen from participating in oxidation reactions (Sathivel et al., 2007).
The amount of TCA-soluble peptides increased during storage, indicating fish protein autolysis. The increase in TCA-soluble peptides may have initially been caused by the activity of endogenous enzymes. Subsequently, protein degradation was accelerated under the combined action of endogenous enzymes and microorganisms (Ge et al., 2016). In this study, TCA-soluble peptide concentration increased sharply in the first 4 days and the last 8 days. It is obviously in accordance with the interpretation of this phenomenon. Furthermore, MMW chitosan coating showed lower TCA-soluble peptide content than uncoated ones, suggesting that it effectively delayed protein denaturation during storage.
When the storage time was prolonged, postmortem proteolysis might cause gradual myofibrillar protein fragmentation of houndfish due to the appearance of band A with molecular weights lower than the MHC band and the increase in the intensity of bands I, II, II, IV, and V. This may be due to the degradation of HMW proteins into smaller molecules via protein hydrolysis by endogenous enzymes (Ge et al., 2016). However, there was no noticeable decrease in MHC and actin content. For uncoated fish, the increase and decrease in intensity of band I on days 4 and 18, respectively, incorporated with less intensity of bands A, III, and IV, attributed to the more degraded myofibrillar protein. In contrast, on day 18, it was observed that the HMW proteins of the coated fish, especially the 1.5% and 2% chitosan samples, tended to show high band intensities. The SDS-PAGE results were in agreement with those of TCA-soluble peptides. This suggested that fish coated with MMW chitosan were less prone to proteolytic degradation.
On the last day of ice storage, all treatments had TVC values lower than the upper limit of acceptability for fresh fish of 7 Log10 CFU/g (ICMSF, 1986). On the other hand, Özogul et al. (2004) reported that when TVC in a food product reaches 106 CFU/g, it is supposed to be at, or near, spoilage. Compared with the latter, only the two controls exceeded 6 Log10 CFU/g on day 16. Regarding microbial safety, the coated samples showed an increase in storage duration of 4 days compared to the control samples. Besides the four antibacterial mechanisms mentioned above (part of Antibacterial activity of MMW chitosan), Priyadarshi & Rhim (2020) reported that chitosan layer formation, similar to cellophane presence on food surfaces, physically prevents microbial attacks and limits gas exchange, inhibiting oxygen transportation and eliminating aerobic microbes. Therefore, chitosan coatings have been shown to reduce microbial growth in seafood products (Yang et al., 2019). Treatments with 1.5% and 2% MMW chitosan coating effectively retarded microbial growth to extend the storage time to 20 days while still maintaining good organoleptic properties. The results of this study are similar to the research of (Fan et al., 2009), which extended the shelf life to 30 days for whole silver carp coated with 2% chitosan compared to only 20 days for uncoated fish when stored at –3°C.
The discoloration in the fish muscle of the control was probably due to several biochemical, chemical, and microbiological changes during fish handling and storage (Chaijan et al., 2005). It was reported that pigments such as myoglobin and hemoglobin are responsible for the color characteristics of fish flesh and are easily oxidized by lipid oxidation products (Yarnpakdee et al., 2012). Lipid oxidation causes yellow discoloration in fish muscle products due to carbonyl groups (Yarnpakdee et al., 2012). This study confirmed the positive effect of 1.5% and 2% MMW chitosan coating treatments against color changes regarding the values of the color lightness and the total color change. Similarly, the lightness of fish meat has been protectively impacted by chitosan coating on tilapia fillets, catfish, and snakehead fish, as demonstrated in previous studies (Yang et al., 2019).
Sensory scores are acceptable for humans and should be evaluated for fish quality and freshness. On the first day of storage, there was no difference in sensory scores, proving that chitosan does not affect the initial sensory attributes of houndfish. The two control samples had sensory scores below the acceptable level after 16 storage days due to strong oxidation, microbial growth, and formed undesirable products negatively affecting appearance, taste, odor, and soft texture (Bazargani-Gilani et al., 2015). In contrast, high sensory scores of the 1.5% and 2% MMW chitosan coating treatments performed during storage showed their maintaining effect on fish quality.
In conclusion, coating treatment of 1.5% and 2% MMW chitosan demonstrated effective quality maintenance and shelf-life extension of ice-stored houndfish for 20 days by significantly reducing lipid oxidation, protein degradation, and microbial growth compared to uncoated samples. This work again confirms that the MMW chitosan coating is entirely suitable for preserving skin-on fish because its natural protective coating almost does not affect flavor, odor, or texture.