Introduction
Sarcopenia, characterized by a decline in muscle mass and function, is known to increase with age (Dodds et al., 2014). Currently, more than 10% of elderly individuals reportedly experience sarcopenia (Ethgen et al., 2017). In Europe, the prevalence of sarcopenia is expected to increase by approximately 72.4% between 65 and 100 years of age by 2045 (Ethgen et al., 2017). Although the mechanism underlying the pathogenesis of sarcopenia needs to be comprehensively elucidated, abnormalities in immune regulation or chronic inflammation are considered to be the main pathophysiology of sarcopenia (Afzali et al., 2018). The regenerative ability of skeletal muscles, muscle function, and muscle mass reportedly decrease during the aging process (Jang et al., 2011). Skeletal muscle consists of post-mitotic and multinucleated cells; thus, these cells typically remain in a quiescent state. However, muscle injuries induce a robust regeneration response, and satellite cells that are committed and self-renewable precursors undergo activation, proliferation, and differentiation (Jang et al., 2011). With aging, satellite cell numbers and functions decrease, associating with declining muscle generation ability (Jang et al., 2011).
Various immune cells play a role in muscle regeneration. Cluster of differentiation (CD) 4+forkhead box P3 (FoxP3)+ regulatory T cells (Tregs) are the dominant T cell subset infiltrating skeletal muscles. Notably, CD4+FoxP3+ Tregs account for 50% of CD+ T cells in the muscle and control inflammation during muscle repair (Burzyn et al., 2013). During muscle repair, the interleukin (IL)-33 signaling pathway is involved in Treg function. IL-33 binds to its receptor ST2 (also known as IL-1 receptor-like 1) and increases Treg proliferation (Kuswanto et al., 2016). IL-33 is primarily secreted by epithelial cells; however, M2 macrophages may also secrete IL-33 (Furukawa et al., 2017).
Tregs play a role in macrophage M2 polarization and increase IL-10 secretion during muscle repair (Burzyn et al., 2013; Deng et al., 2012). Both M1 and M2 macrophages mediate essential functions during muscle repair. Immediately after muscle injury, M1 macrophages, known to exhibit pro-inflammatory characteristics, appear and trigger satellite cell proliferation (Arnold et al., 2007). Subsequently, M1 macrophages shift to M2 macrophages for mediating muscle regeneration. M2 macrophages exhibit anti-inflammatory characteristics and enhance myoblast fusion and myotube hypertrophy (Arnold et al., 2007).
IL-10 is essential for skeletal muscle repair (Deng et al., 2012). Tregs in skeletal muscle can secrete both IL-10 and the epidermal growth factor-like ligand amphiregulin (Areg), especially after sterile muscle injury (Burzyn et al., 2013). Co-culture with Tregs enhances satellite cell proliferation (Castiglioni et al., 2015) Areg, which is secreted by muscle Tregs, directly acts on satellite cells and triggers muscle regeneration (Burzyn et al., 2013). The essential role of Areg-producing Tregs for muscle regeneration was demonstrated in an animal model with specific ablation of Treg cells (Arpaia et al., 2015).
Collectively, Tregs can play various roles in regulating muscle inflammation and satellite cell function during muscle regeneration. Aging decreases Treg-mediated immunological regulation during muscle regeneration (Domingues-Faria et al., 2016). Furthermore, Treg infiltration in aged muscles is reduced due to various factors related to Treg population dynamics, including Treg recruitment, proliferation, and retention (Kuswanto et al., 2016). Autophagy is a crucial regulator of Treg stability and survival (Wei et al., 2016). Autophagy-related (ATG) proteins play an essential role during autophagy as the ‘core machinery’ of autophagy (Yang et al., 2010). Mice with specific deletion of ATG5 in Treg cells (Foxp3CreAtg5fl/fl) reportedly demonstrate disordered homeostasis in CD4+ and CD8+ cells. The percentage of Tregs decreased while interferon (IFN)-γ expression increased (Wei et al., 2016).
The Crassostrea gigas oyster is a widely cultivated, industrially important shellfish in Asia because of its low impact on the natural environment and high sustainability. Furthermore, oyster meat is a great source of vitamins and essential minerals (such as zinc, iron, calcium, and selenium), and, especially glutamic acid, a precursor of γ-aminobutyric acid (GABA) (Bang et al., 2020). GABA is a well-known inhibitory neurotransmitter of the central nervous system that can act as an immunomodulatory molecule has known to affect several functions in the body, including muscle health.
The fermentation process has been known to change the biochemical component of the substance including change in the structure of amino acid (AA), and, hence altering its biological activities (Molagoda et al., 2022). AAs play an important biological and chemical role in the body, especially in building and repairing tissues. Especially, a special class of AAs known as branched-chain amino acids (BCAAs; leucine, isoleucine, and valine) has been used as a substrate for protein synthesis and can stimulate skeletal muscle synthesis and suppress proteolysis (Mann et al., 2021). Delay of AAs absorption, anabolic resistance, and low level of BCAAs are common in the elderly and are associated with the declination of muscle mass, strength, and function (Ko et al., 2020). Studies reported that supplementary BCAAs and essential AAs can attenuate sarcopenia in the elderly and stimulate muscle protein synthesis (ter Borg et al., 2019).
Our previous studies reported that that fermentation of oysters by Lactobacillus brevis BJ20 increased the amount of GABA, and lactic acid, and had a positive effect on bone formation (Molagoda et al., 2022), height growth (Jeong et al., 2021) and muscle endurance (Reid et al., 2020). Recently, we demonstrated that GABA–enriched fermented sarco oyster extract (FSO) reduced muscle proteolysis by increasing mRNA levels of insulin-like growth factor (IGF)-1 in dexamethasone-induced animals (Oh et al., 2021). Furthermore, GABA, as an immunomodulatory molecule can decrease pro-inflammatory cytokine production (Mazzoli & Pessione, 2016). Autophagy mediates immune regulation through GABA regulation. During autophagy, GABA upregulates ATG5 in the mesenchymal lymph node cells (MLNCs), decreasing the production of IFN-γ and IL-17 by MLNCs (Bajić et al., 2020), and also upregulates the expression of Foxp3+, IL-10, and transforming growth factor-β (TGF-β) in MLNCs (Bajić et al., 2020). Respectively, T helper (Th) 17 and Th1 cells secrete IL-17 and IFN-γ induces pro-inflammatory responses (Kuwabara et al., 2017) and by secreting anti-inflammatory cytokines such as IL-10 and TGF-β (Dominguez-Villar & Hafler, 2018), Tregs reduces the inflammatory response.
Although studies have shown that FSO (with high GABA and lactate content) can enhance muscle health and attenuated muscle atrophy (Oh et al., 2021), the modulation process of GABA in FSO in relation to increase in Treg in aged muscle is still unknown. The aim of this study is to determine (1) the impact of fermentation process in FSO in relation to biochemical components, especially protein-related BCAAs, and, (2) the impact of FSO on autophagy and Tregs and its modulating ability in satellite cells regeneration. Here, we used aged mice to compare the effect of FSO on muscle young mice and aged mice treated with GABA+Lactic acid administration. We hypothesized that the fermentation process results in an increase in BCAAs and lower molecular weight AA for easy absorption and FSO enhances autophagy and Tregs, which decreases IL-17 and IFN-γ and increase IL-10 and TGF-β. Furthermore, increased Tregs can also lead to elevated IL-33 levels, eventually enhancing M2 polarization.
Materials and Methods
In this study, the FSO used was sourced from Marine Bioprocess (Busan, Korea) and prepared as follows. Frozen and peeled oysters purchased from Deokyeon Seafood (Tongyeong, Korea) were washed, drained, and homogenized. The homogenized oysters were hydrolyzed with alcalase (Alcalase® 2.4 L, FG, Brenntag Korea, Seoul, Korea) at 60 ± 5°C for 4 hours, then filtered with a vibrating sieve (120 mesh, Buchi Labortechnik GmbH, Essen, Germany), and concentrated using a rotary evaporator (Buchi Labortechnik GmbH).
To manufacture the FSO, L. brevis BJ20 (accession no. KCTC 11377BP) and Lactobacillus plantarum BJ21 (accession no. KCTC18911P) were separately inoculated into sterilized (121°C, 15 min) seed medium (yeast extract 3%, glucose 1%, monosodium glutamate 1%, water 95%) and cultured at 37°C for 24 hours. Next, 10% (v/v) of the L. brevis BJ20 cultured seed medium was fermented in a fermentation medium (yeast extract 2%, glucose 2%, L-glutamic acid 5%, hydrolyzed oyster extract 50%, water 41%) at 37°C for 24 hours. After that, 10% (v/v) of the L. plantarum BJ21 cultured seed medium was added to the previous medium and fermented at 37°C for 24 hours. The fermentation medium was filtered (disk separator, Alfa Laval, CLARA 200), concentrated, and spray-dried to prepare powder sample FSO, which contained GABA (11.2%), lactic acid (5.0%), water (5.5%), protein (31.8%), and carbohydrate (56.1%) (Oh et al., 2021).
GABA, L-(+)-lactic acid, and sodium acetate (50 mM, pH 6.5) were purchased from Sigma-Aldrich (St. Louis, MO, USA). Acetonitrile, Methanol, and distilled water were high-performance liquid chromatography (HPLC)-grade and were purchased from Samchun Pure Chemical (Pyeongtaek, Korea). The hydrochloric acid solution was purchased from Biosesang (Seongnam, Korea). OPA regent (10 mg/mL, Agilent P/N 5061-3335) and borate buffer (0.4 N in water, pH 10.2; Agilent P/N 5061-3339) were purchased from Agilent Technologies (Palo Alto, CA, USA). Acetic acid, monopotassium phosphate, and phosphoric acid were purchased from Junsei Chemical (Tokyo, Japan).
L-(+)-lactic acid and GABA stock solution were prepared by dissolving 0.1 g of each chemical with 100 mL of distilled water in a volumetric flask. Solutions were filtered through PTFE Syringe Filter (Lbscience, Busan, Korea), 25 mm/0.2 μm to remove small particles. The solutions were kept at –80°C. For the 5% aqueous sample solution, 5 g of FSO was dissolved in a 100 mL volumetric flask with distilled water. For lactic acid analysis, the sample solution was diluted 20X with distilled water and for GABA analysis, the sample solution was diluted 20X with 0.02 M HCl aqueous solution. Each solution was filtered through PTFE Syringe Filter, 25 mm/0.2 μm.
GABA content was determined using a HPLC system (Dionex U3000; Thermo Fisher Scientific, Sunnyvale, CA, USA) equipped with a UV detector. Dionex bonded silica column (C18, 5 μm 120 Å 4.6 × 50 mm) fitted with 4.0 × 3.0 mm i.d. guard column, both from Phenomenex (Torrance, CA, USA), were used. Solvent A was 50 mM sodium acetate (pH 6.5), and solvent B consisted of 45% (v/v) acetonitrile, 45% (v/v) methanol, and 10% (v/v) distilled water. The linear gradient was conducted for 30 min at 338 nm with an injection volume of 20 µL.
Lactic acid content was determined using a HPLC system (Dionex U3000) equipped with a UV detector. Dionex bonded silica column (C18 5μm 120 Å 4.6 × 250 mm) fitted with 4.0 × 3.0 mm i.d. guard column, both from Phenomenex, were used. For isolation condition analysis, solvent A was 0.2 M potassium phosphate (pH 2.4). The isocratic was conducted for 15 min at 214 nm and the injection volume was 20 µL. The solvent flow rate was 0.8 mL/min. The colunm temperature during the analysis was kept at 40°C.
The AA analysis of FSO to determine and compare the content of protein-bound amino acids (PAAs) and FAAs; pre-and post-fermentation was accomplished by Pukyong National University (Busan, Korea).
We obtained male and female C57BL/6N mice, aged six weeks, from Orient Bio (Seoul, Korea). After the adaptation period, the males and females were paired for mating, and their offspring were observed for either 4 or 12 months. The male mice that were raised for 4 months were categorized as the young group (n = 5), while the 12-month-old males were randomly assigned to five aging groups (n = 5 per group). In total, the mice were divided into six groups.
-
1) Young/Saline: Saline was orally administered to 4-month-old animals.
-
2) Aging/Saline: Saline was orally administered to 12-month-old animals.
-
3) Aging/FSO50: FSO 50 mg/kg/day was orally administered to 12-month-old animals.
-
4) Aging/FSO100: FSO 100 mg/kg/day was orally administered to 12-month-old animals.
-
5) Aging/FSO200: FSO 200 mg/kg/day was orally administered to 12-month-old animals.
-
6) Aging/GABA+Lactic acid: GABA at 5 mg/kg/day and lactic acid at 4 mg/kg/day were orally administered to 12-month-old animals.
Oral administration was performed for four weeks, and a grip strength test was subsequently performed. All animal experiments were approved and performed according to the ethical principles of the Institutional Animal Care and Use Committee of Gachon University (approval no. LCDI-2021-0074).
Following a 4-week period of oral administration, we assessed the grip strength of mice using a grip strength meter (Jeungdo Bio & Plant, Seoul, Korea). The grip strength meter provides a digital readout of the maximum force. To measure the grip strength, the mouse was placed on the meter bar, and its tail was gently pulled. We performed ten measurements at 30 s intervals and calculated the average grip strength.
We collected muscles from mice and preserved them by immersing them in 4% paraformaldehyde (Sigma-Aldrich) dissolved in cold phosphate-buffered saline (PBS; Biosesang) for 24 h at 4°C. The fixed muscles were washed with tap water for 1 h and then processed for dehydration and wax infiltration using a tissue processor (Sakura Finetek, Tokyo, Japan). Finally, the tissues were embedded in the direction of the cross-sectional area (CSA) using an embedding machine (Sakura Finetek).
To extract proteins from frozen muscles, we lysed 50 mg of muscle tissue with 0.5 mL of radioimmunoprecipitation assay lysis buffer containing protease and phosphatase inhibitors (ATTO, Tokyo, Japan). The tissue was then homogenized using a bead homogenizer (Allsheng Instrument, Hangzhou, China) at 6.0 m/s for 10 cycles (running for 40 s and interrupting for 60 s). After sonication, the homogenized tissue was centrifuged at 14,000×g for 20 min at 4°C. The supernatant was transferred to a new tube and quantified using a bicinchoninic acid assay kit (Thermo Fisher Scientific).
The total RNA was extracted following the manufacturer’s instructions. To summarize, we added 100 mg of frozen muscle to 1 mL RNAiso (Takara, Tokyo, Japan) and homogenized it at 6.0 m/s for 10 cycles (running for 40 s, interrupted for 60 s) using a bead homogenizer (Allsheng Instrument). After incubating the homogenized tissue for 5 min at room temperature and centrifuging it at 12,000×g for 5 min at 4°C, the supernatant was transferred to a new tube. Then, we added 0.2 mL of chloroform, vigorously vortexed the mixture, and incubated it for 5 min at room temperature, followed by centrifugation at 12,000×g for 15 min at 4°C. Afterward, we transferred the upper layer to a new tube, added 0.5 mL of isopropanol, incubated it for 10 min at room temperature, and centrifuged it at 12,000×g for 10 min at 4°C. We discarded the solution, added 1 mL of 75% ethanol, and centrifuged it at 7,000×g for 5 min at 4°C. Then, we discarded the solution, leaving only the RNA pellet, which was air-dried and dissolved in 50 µL diethyl pyrocarbonate-treated water.
We followed the manufacturer’s protocol to synthesize complementary DNA (cDNA) using the PrimeScript First Strand cDNA Synthesis Kit (Takara). To summarize, RNA was quantified using a Nanodrop (Thermo Scientific), and 1 µg of RNA was added to a new tube. Then, 1 µL of Oligo dT Primer, 1 µL of dNTP mixture, and RNase-free dH2O were added to bring the total volume to 10 µL. The solution was incubated for 5 min at 65°C and then cooled on ice. Next, 4 µL of 5X PrimeScript Buffer, 0.5 µL of RNase Inhibitor, 1 µL of PrimeScript RTase, and 4.5 µL of RNase-free dH2O were added to the sample to achieve a final volume of 20 µL. The mixture was then incubated for 45 min at 42°C, followed by 5 min at 95°C, and then cooled on ice.
The paraffin-embedded muscle blocks were sliced into 7 µm thick sections and placed on silane-coated slides (Muto Pure Chemical, Tokyo, Japan) and left to dry for 24 h at 60°C in a slide warmer (JISICO, Seoul, Korea). The slides were deparaffinized and incubated with normal animal serum to prevent non-specific antibody binding. Primary antibodies (Supplementary Table S1) were applied to the tissues at 4°C overnight, and rinsed three times with PBS. Subsequently, secondary antibodies (Supplementary Table S1) were added for 1 hour at room temperature, followed by three PBS rinses. The tissues were then counterstained with 4`,6-diamidino-2-phenylindole (Sigma-Aldrich) and mounted with Vectashield mounting medium (Vector Laboratories, Burlingame, CA, USA). Stained tissue images were captured using a confocal microscope (LSM 710, Carl Zeiss, Oberkochen, Germany) at the Core Facility for Cell to In-Vivo Imaging (Incheon, Korea) and images were analyzed with the Zen 2009 software (Carl Zeiss).
The slides were deparaffinized and blocked with normal animal serum to prevent non-specific antibody binding. Tissues were incubated with primary antibodies (Supplementary Table S1) overnight at 4°C and rinsed thrice with PBS. Then, the tissues were treated with biotinylated secondary antibodies (Supplementary Table S1) for 1 h at room temperature and rinsed thrice with PBS. The tissues were then incubated with ABC reagent (Vector Laboratories) for 30 min, rinsed thrice with PBS, and reacted with 3,3-diaminobenzidine (DAB) substrate for 2 min. The tissues were finally mounted with a distyrene–plasticizer–xylene mounting solution (DPX; Sigma-Aldrich). Images of stained tissues were captured using a light microscope (Olympus, Tokyo, Japan), and the intensity of the brown color was quantified using ImageJ software (National Institutes of Health, Bethesda, MD, USA).
The slides were then deparaffinized and stained with hematoxylin (Dako, Glostrup, Denmark) and eosin Y (Sigma-Aldrich) for 30 s each. The stained tissues were mounted with DPX (Sigma-Aldrich). Images of stained tissues were captured using a light microscope (Olympus), and the mean fiber CSA was quantified using ImageJ software (National Institutes of Health).
The 30 μg of protein was isolated and separated on 8%–12% polyacrylamide gels, followed by transfer onto polyvinylidene fluoride membranes (Millipore, Burlington, MA, USA) using a power station (ATTO). Next, the membranes were blocked with 5% skim milk for 1 h and washed with Tris-Buffered Saline containing 0.1% Tween 20 (TTBS). The membranes were then incubated with primary antibodies (Supplementary Table S1) overnight at 4°C and washed three times with TTBS. Following this, peroxidase-conjugated secondary antibodies (Supplementary Table S1) were incubated with the membranes for 1 h at room temperature and washed three times with TTBS. Finally, the membranes were visualized using a western blotting detection reagent (Cytiva, Seoul, Korea).
To perform quantitative reverse transcription-polymerase chain reaction (qRT-PCR), cDNA was prepared and amplified using the CFX384 TouchTM Real-Time PCR detection system (Bio-Rad Laboratories, Hercules, CA, USA). Specifically, 200 ng of cDNA was combined with 5 µL of SYBR premix (Takara) and 0.4 µM forward and reverse primers (Supplementary Table S2), and the threshold cycle numbers were determined using CFX ManagerTM software (Bio-Rad Laboratories).
The data are reported as mean ± SD, and all statistical analyses were conducted using SPSS version 22 (IBM Corporation, Armonk, NY, USA). The Kruskal-Wallis test was utilized for comparing each group, and a post hoc Mann-Whitney U test was conducted to determine statistical significance. In this study, different letters were assigned to groups that showed significant intergroup differences: * vs. Young/Saline; # vs. Aging/Saline; $ vs. Aging/FSO100; † vs. Aging/GABA+Lactic acid.
Results
To validate the content of FSO, the HPLC chromatogram of lactic acid and GABA standard was compared to the FSO sample HPLC analysis (Fig. 1). The mean retention time for standard lactic acid and GABA were 6.56 ± 0.03 min and 12.36 ± 0.06 min. As for FSO, the retention time for lactic acid was 6.53 ± 0.04 min and for GABA was 12.27 ± 0.04 min. Both lactic acid and GABA have a suitable resolution (> 0.5 min) from other acids and AAs in FSO.
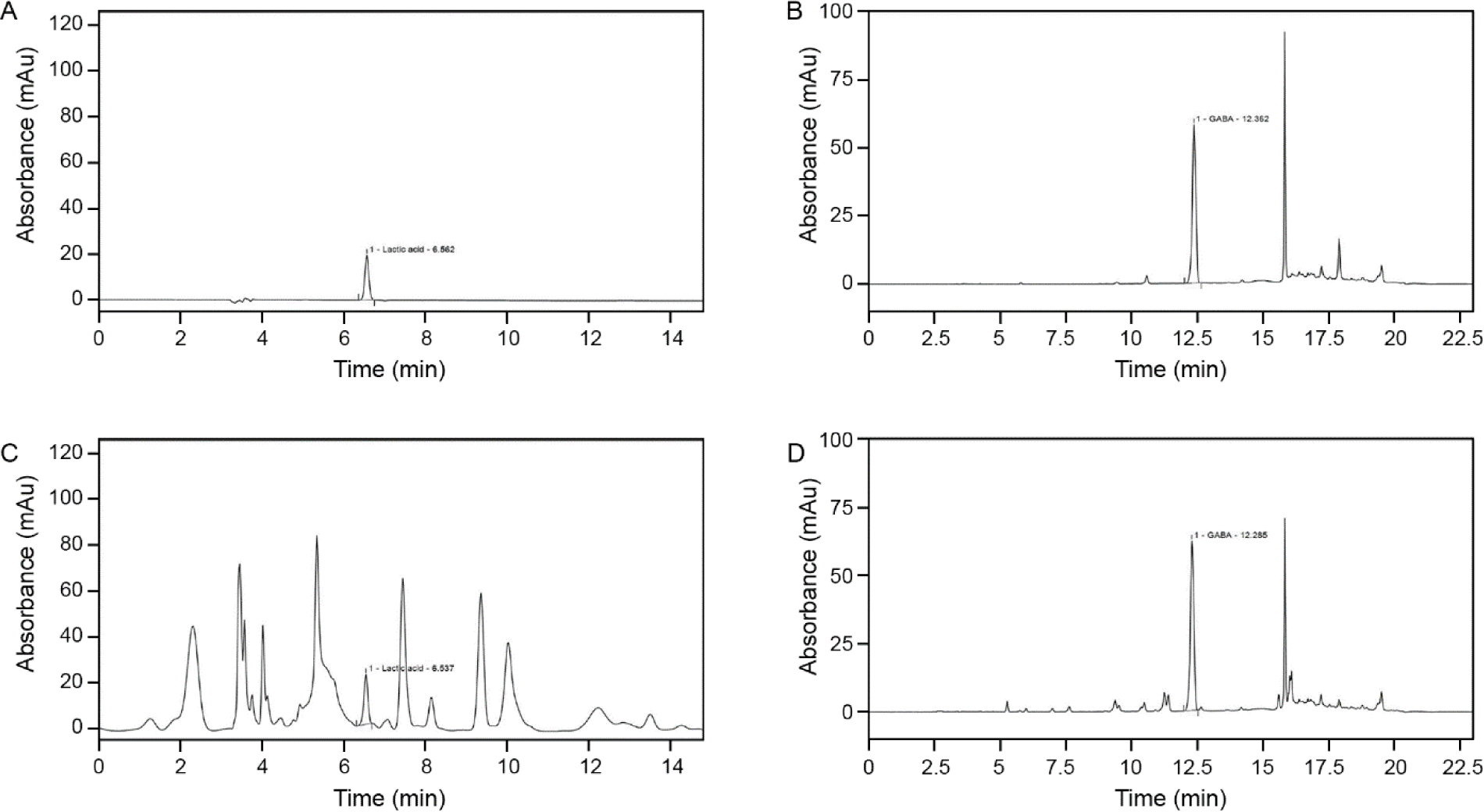
To determine the effect of the fermentation period on GABA and lactic acid content, production-time profile measurements were made at the pre-fermentation point and at 4, 8, 12, 16, 20, 24, 28, and 32 h after fermentation (Table 1). The results showed an increase in both GABA and lactic acid after 4 h of fermentation, and then plateaued after 16 h with GABA and lactic acid content of 10.21 ± 0.19 and 5.02 ± 0.10 with slight fluctuation. The maximum content was achieved after 32 h of fermentation with values of 10.48 ± 0.25 and 5.48 ± 0.11.
The composition of PAAs and FAAs of FSO pre- and post-fermentation are summarized in Tables 2 and 3. The comparison of PAAs composition showed that with the exception of phenylalanine, the rest of PAAs composition decreased, where glutamic acid and proline had the most significant change. As for the composition of FAA, the majority of FAAs, including BCAAs (isoleucine, leucine and valine) increased. Furthermore, in FAAs GABA increased the most, while glutamic acid; the metabolic precursor of GABA decreased the most after fermentation.
We first evaluated whether FSO could increase ATG signals in Tregs. Expression of FoxP3 is essential for establishing Treg cell function during differentiation, as well as for the maintenance of Tregs in the periphery (Rudensky, 2011). Accordingly, we used FoxP3 as a Treg marker. ATG, GABARAP, and Beclin1 are frequently assessed as autophagy markers (Harada et al., 2010). Moreover, the conversion of the soluble cytosolic form of microtubule-associated protein 1A/1B-light chain 3 (LC3) I into the bound LC3II form (LC3II/LC3I) is widely used as an autophagy marker (Ouyang et al., 2010).
We observed that the number of cells co-stained with ATG5 and FoxP3 was significantly lower in aged muscle than in young muscle. However, administration of 50, 100, and 200 mg/kg FSO and GABA+Lactic acid significantly increased the number of ATG5 and FoxP3 stained cells in the aged muscle. In addition, no statistical difference was observed between 100 and 200 mg/kg FSO and GABA+Lactic acid groups (Fig. 2A and 2B).
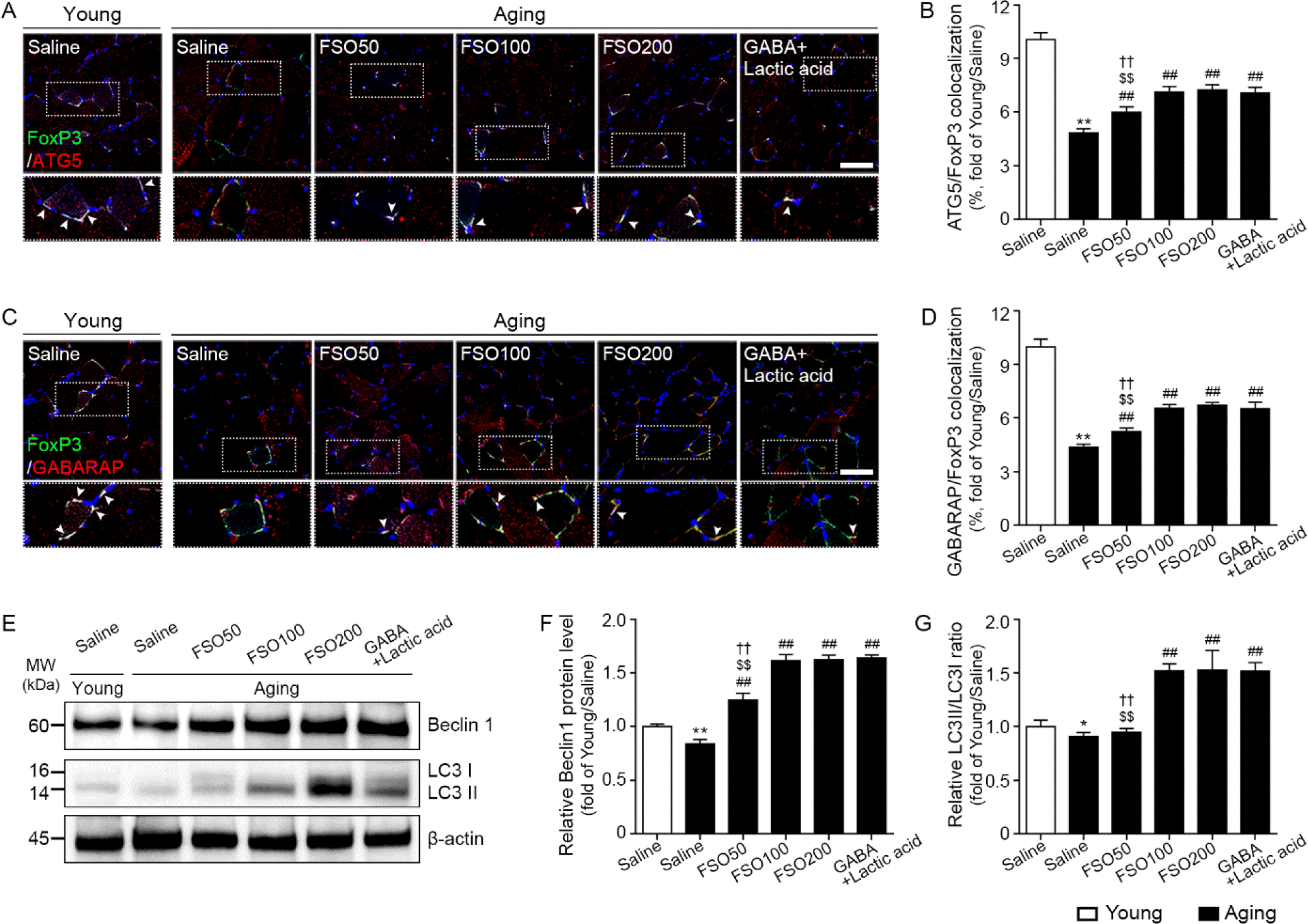
The expression of GABARAP in FoxP3 positive cells was significantly lower in aged muscles than in young muscles. However, administration of 50, 100, and 200 mg/kg FSO and GABA+Lactic acid significantly increased the number of cells co-stained with GABARAP and FoxP3 in the aged muscle. No statistical difference was observed between 100 and 200 mg/kg FSO and GABA+Lactic acid groups (Fig. 2C and 2D).
The expression of Beclin1, evaluated by western blotting, was significantly lower in aged muscles than in young muscles. However, administration of 50, 100, and 200 mg/kg FSO and GABA+Lactic acid significantly increased Beclin1 expression in the aged muscle. No statistical difference was observed between 100 and 200 mg/kg FSO and GABA+Lactic acid groups (Fig. 2E and 2F).
The expression ratio of LC3II/LC3I was significantly lower in aged skeletal muscles than that in young muscles. However, administration of 100 and 200 mg/kg FSO and GABA+Lactic acid significantly increased the ratio of LC3II/LC3I expression in the aged muscle. No statistical difference was observed between 100 and 200 mg/kg FSO and GABA+Lactic acid groups (Fig. 2E and 2G). Thus, these results revealed that FSO increased autophagy in both Tregs and muscles.
We evaluated muscle Treg cell accumulation by determining the mRNA expression of FoxP3. The expression of FoxP3 was significantly lower in aged muscles than in young muscles. However, administration of 50, 100, and 200 mg/kg FSO and GABA+Lactic acid significantly increased FoxP3 expression in aged muscle; this effect increased in a dose-dependent manner in the FSO group. No statistically significant difference was observed between the 100 and 200 mg/kg FSO and GABA+Lactic acid groups (Supplementary Fig. S1A).
Next, we evaluated whether increased Tregs led to elevated levels of anti-inflammatory cytokines (TGF-β and IL-10) and decreased levels of proinflammatory cytokines (IFN-γ and IL-17A) in the muscle.
The mRNA expression of TGF-β was significantly lower in aged muscles than in young muscles. Administration of 50, 100, and 200 mg/kg FSO and GABA+Lactic acid increased the expression of TGF-β in the aged muscle; this effect increased in a dose-dependent manner in the FSO group. No statistically significant difference was observed between 100 and 200 mg/kg FSO and GABA+Lactic acid groups (Supplementary Fig. S1B).
The mRNA expression of IL-10 was significantly lower in aged muscles than in young muscles. Administration of 50, 100, and 200 mg/kg FSO and GABA+Lactic acid increased IL-10 expression in the aged muscle. The effect increased in a dose-dependent manner in the FSO group. Moreover, the 200 mg/kg FSO group demonstrated a superior effect (Supplementary Fig. S1C).
The mRNA expression of IFN-γ was significantly higher in aged muscles than in young muscles. Administration of 50, 100, and 200 mg/kg FSO and GABA+Lactic acid decreased IFN-γ expression; this effect did not significantly differ between 100 and 200 mg/kg FSO and GABA+Lactic acid groups (Supplementary Fig. S1D).
The mRNA expression of IL-17A was significantly higher in aged muscles than in young muscles. Administration of 50, 100, and 200 mg/kg FSO and GABA+Lactic acid de-creased IL-17A expression; this effect did not significantly differ between 100 and 200 mg/kg FSO and GABA+Lactic acid groups (Supplementary Fig. S1E).
We determined whether increased Tregs enhanced Treg function for muscle regeneration. Accordingly, IL-33, ST2, and Areg expression levels were determined using immunohistochemical staining or mRNA expression.
The expression of IL-33 was significantly decreased in aged muscle when compared with that in young muscle. Administration of 50, 100, and 200 mg/kg FSO and GABA+Lactic acid significantly increased expression, with a dose-dependent increase observed in the FSO group. Moreover, the 200 mg/kg FSO group demonstrated a superior effect (Fig. 3A and 3B).
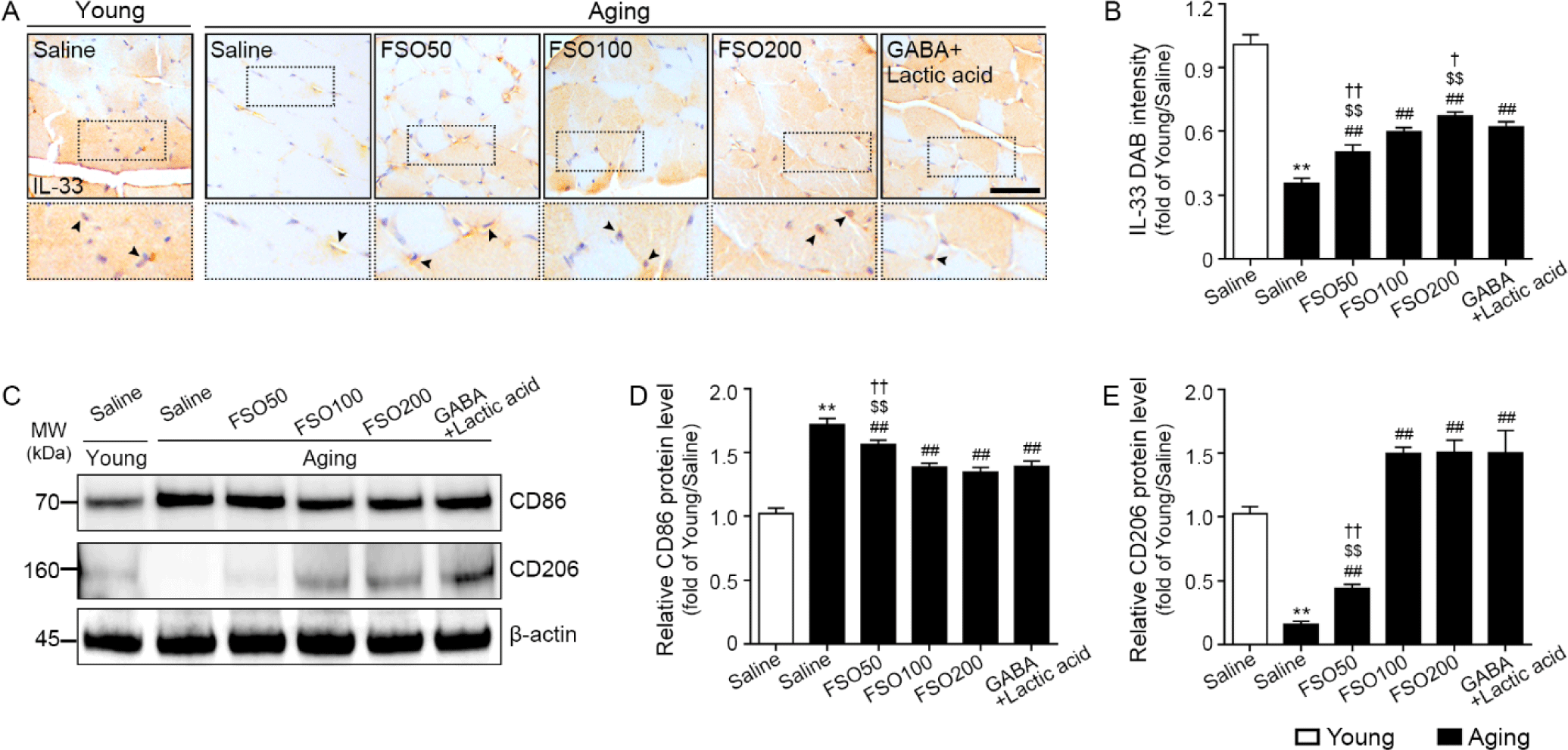
The expression of ST2 was significantly decreased in aged muscle when compared with young muscle. Administration of 50, 100, and 200 mg/kg FSO and GABA+Lactic acid significantly increased ST2 expression, with a dose-dependent increase observed in the FSO group. The increasing effect of GABA+lactic acid was significantly higher than that of 50 mg/kg FSO and was significantly lower than that of 200 mg/kg FSO. No statistically significant difference was observed between the FSO 100 mg/kg and GABA+lactic acid groups (Supplementary Fig. S2A).
Areg expression was significantly decreased in aged muscle when compared with young muscle. Administration of 100 and 200 mg/kg FSO and GABA+Lactic acid significantly increased Areg expression; the increasing effects of 100 mg/kg and 200 mg/kg FSO and GABA+Lactic acid did not differ significantly (Supplementary Fig. S2B).
To determine whether increased Tregs led to M2 polarization, we performed western blotting for CD86 (M1 marker) and CD206 (M2 marker).
The expression of CD86 was significantly higher in aged muscles than in young muscles. Administration of 50, 100, and 200 mg/kg FSO and GABA+Lactic acid decreased CD86 expression. The decreasing effects of 100 mg/kg and 200 mg/kg FSO and GABA+Lactic acid did not differ significantly (Fig. 3C and 3D).
The expression of CD206 was significantly lower in aged muscles than in young muscles. Administration of 50, 100, and 200 mg/kg FSO and GABA+Lactic acid increased CD206 expression. The increasing effects of 100 mg/kg and 200 mg/kg FSO and GABA+Lactic acid did not differ significantly (Fig. 3C and 3E).
To examine satellite cell accumulation in muscle, we examined the expression of PAX7, a satellite cell marker (Sambasivan et al., 2011). The expression of PAX7 was significantly lower in aged muscles than in young muscles. Administration of 100 and 200 mg/kg FSO, and GABA+Lactic acid increased PAX7 expression. Moreover, the 200 mg/kg FSO group demonstrated a superior effect (Fig. 4A and 4B).
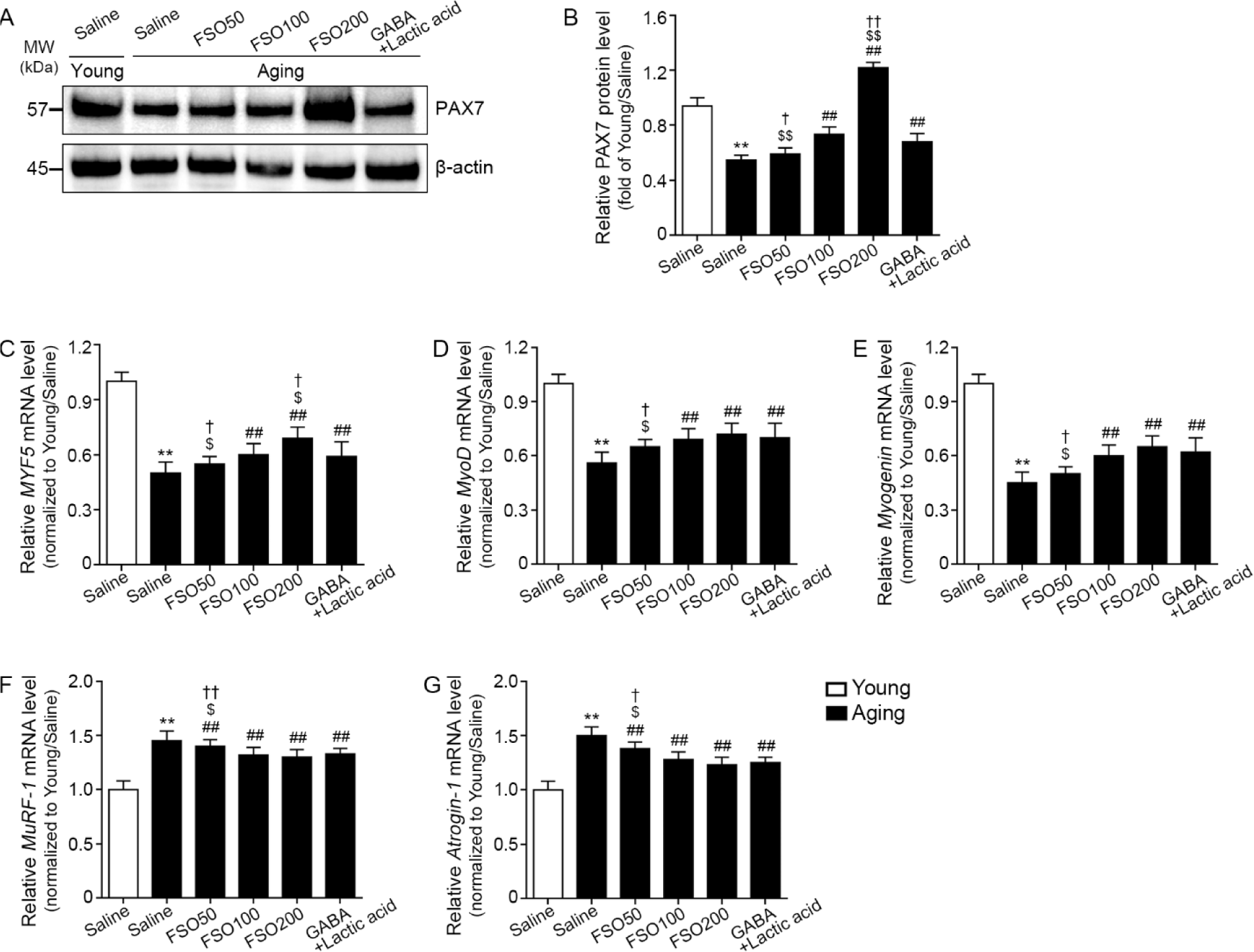
We evaluated muscle expression of myogenic determination gene number 1 (MyoD), myogenin, and myogenic factor 5 (MYF5), well-known regenerative myogenesis markers (Zammit, 2017). The expression of MYF5 was significantly decreased in aged muscle when compared with that in young muscle; administration of 100 mg/kg and 200 mg/kg FSO and GABA+Lactic acid increased the expression of this marker. Moreover, the 200 mg/kg FSO group demonstrated a superior effect (Fig. 4C).
MyoD expression was significantly decreased in aged muscle when compared with that in young muscle. Administration of 100 mg/kg and 200 mg/kg FSO and GABA+Lactic acid increased MyoD expression. The increasing effects of 100 mg/kg and 200 mg/kg FSO and GABA+Lactic acid did not differ significantly (Fig. 4D).
Compared with young muscle, myogenin expression was significantly decreased in aged muscle. Administration of 100 mg/kg and 200 mg/kg FSO and GABA+Lactic acid increased myogenin expression. The increasing effects of 100 mg/kg and 200 mg/kg FSO and GABA+Lactic acid did not differ significantly (Fig. 4E).
MuRF-1 and atrogin-1 are E3 ubiquitin ligases that lead to proteolysis in skeletal muscles (Bodine et al., 2001). Compared with young muscle, expression levels of MuRF-1 and atrogin-1 were significantly increased in aged muscle; administration of 50, 100, and 200 mg/kg FSO and GABA+Lactic acid decreased expression levels. The effects mediated by 100 mg/kg and 200 mg/kg FSO and GABA+Lactic acid did not differ significantly (Fig. 4F and 4G).
The gastrocnemius muscle mass was evaluated using the muscle weight (normalized by body weight), the thickest diameter of the gastrocnemius muscle, and mean muscle fiber CSA.
Muscle weight, which was normalized by body weight, was significantly lower in aged muscle than in young muscle. Administration of 50, 100, and 200 mg/kg FSO and GABA+Lactic acid increased muscle weight. The effects of 100 mg/kg and 200 mg/kg FSO and GABA+Lactic acid did not differ significantly (Fig. 5A and 5B).
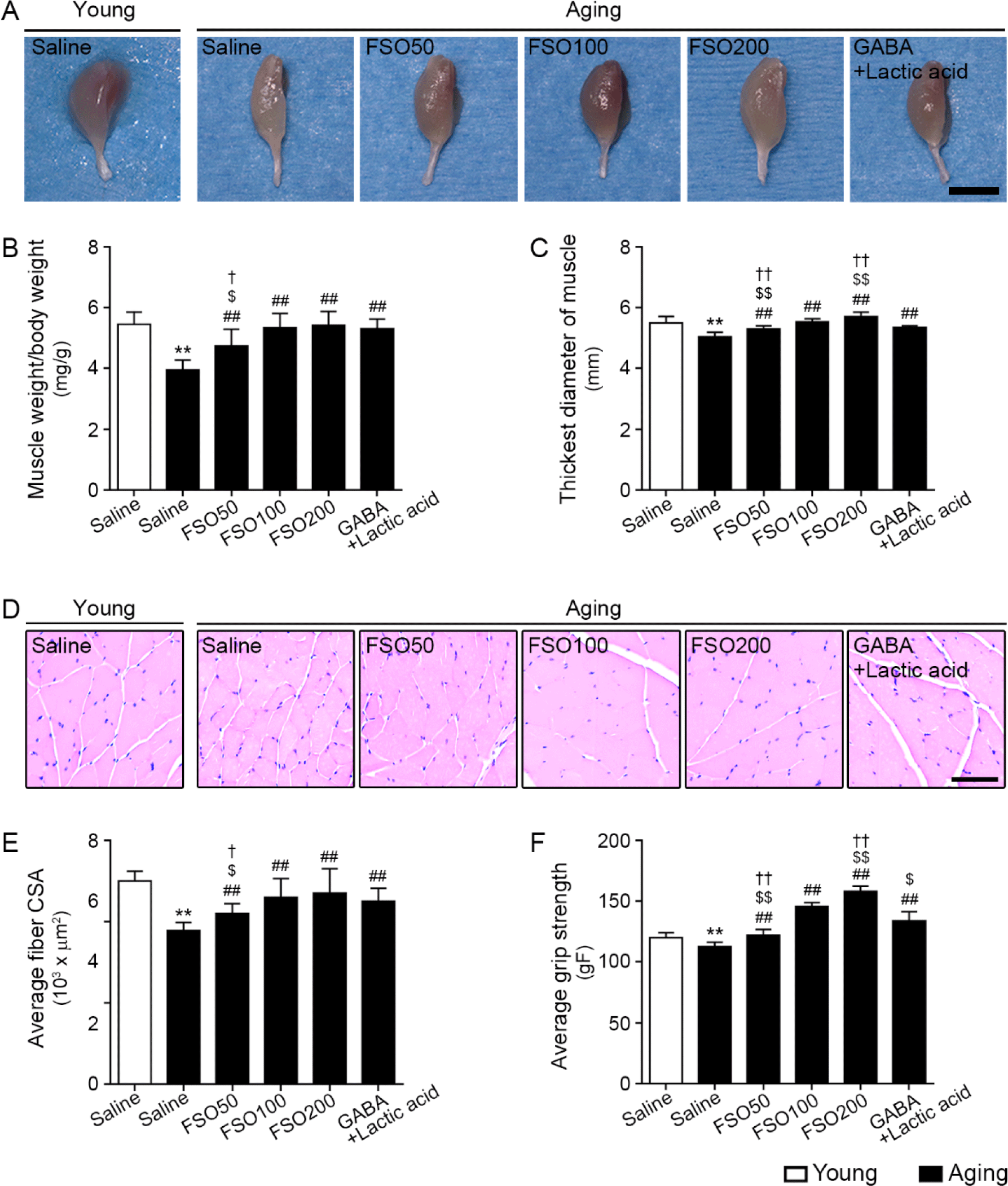
The thickest muscle diameter was significantly lower in aged muscle than in young muscle; administration of 50, 100, and 200 mg/kg FSO and GABA+Lactic acid increased this parameter, with the 200 mg/kg FSO group demonstrating a superior effect (Fig. 5A and 5C).
The mean muscle fiber CSA was significantly lower in aged muscle than in young muscle. Administration of 50, 100, and 200 mg/kg FSO and GABA+Lactic acid increased muscle fiber CSA. The increasing effects of 100 mg/kg and 200 mg/kg FSO and GABA+Lactic acid did not differ significantly (Fig. 5D and 5E).
Grip strength was significantly lower in aged muscles than in young muscles. Administration of 50, 100, and 200 mg/kg FSO and GABA+Lactic acid increased grip strength; the 200 mg/kg FSO group exhibited the most prominent effect (Fig. 5F).
Discussion
GABA leads to an increase in ATG5-dependent autophagy, which eventually results in Treg function modulation and decreases pro-inflammatory cytokine levels (Bhat et al., 2010). GABA increases the expression of ATG signals, such as Unc51-like kinase 1 (ULK1), Beclin1, ATG5, LC3, and GABARAP, in MLNCs (Bhat et al., 2010). Moreover, GABA can decrease the expression levels of IL-17A and IFN-γ in MLNCs, while increasing those of IL-10 and TGF-β (Bhat et al., 2010). It is well-known that Tregs play a role in muscle regeneration by modulating inflammation and satellite cell function (Burzyn et al., 2013). Thus, we evaluated whether GABA-enriched FSO enhanced Treg function by increasing autophagy.
Fermentation is a degrading process, known to contain higher nutritious value than unfermented counterparts (Cui et al., 2020). Studies have shown that fermentation can impact the content and composition of PAAs, FAAs, GABA, and lactic acid (Cui et al., 2020). First, we evaluated the effect of fermentation on PAAs and FAAs. The results showed that after fermentation, the composition of PAAs decreased while majority of FAAs increased, including BCAA’s which are water soluble and shorter, hence easier to digest and to be absorbed. Glutamic acid is a precursor of GABA and lactic acid bacteria are one of the most effective GABA producers and have been known to aid in muscle fatigue and regeneration (Cui et al., 2020). The results showed that fermentation resulted in 70 percent GABA conversion, which coincides with our pilot study where fermentation has a significant influence on the conversion glutamic acid into GABA. For further confirmation of the fermentation effect in GABA and lactic acid production, both concentrations were measured by fermentation time increments. The results showed that within 8 hours of fermentation, the concentration of lactic acid and GABA increased, and after 16 hours of fermentation the concentration reached its peak. To ensure its maximum concentration, fermentation was prolonged to a total fermentation process of 48 hours, and the maximum amount of lactic acid and GABA were confirmed in the present study. Both analytic results showed in GABA but the concentration did differ. However, the sample lot for quantitative analysis of PAA, and FAA was different from the sample for the change in PAA and FAA before and after fermentation was different. Considering that difference in value is within the validation range of 80%–120%, both measurements can be considered valid.
The accumulation of ATG5-positive FoxP3+ cells was reduced in aged muscles. In addition, GABARAP-positive FoxP3+ cell accumulation was decreased in aged muscles. FSO administration increased both ATG5- and GABARAP-positive FoxP3+ cells, suggesting that FSO upregulated autophagy signals in Tregs, associated with Treg function. Moreover, ATG signals, including Beclin1 and LC3II/LC3I, were reduced in aged muscle, and FSO administration enhanced these signals.
Tregs exhibit potent anti-inflammatory effects. Thus, decreased Treg numbers or function in aged muscles can aggravate the catabolic effect of inflammation (Saini et al., 2016). In the present study, Treg accumulation, evaluated by determining FoxP3 expression, was decreased in aged muscle, which was increased following FSO treatment. The anti-inflammatory function of Tregs was evaluated by determining the expression of anti-inflammatory cytokines in the muscle. Expression levels of anti-inflammatory cytokines, including IL-10 and TGF-β, were significantly decreased in the aged muscle; however, these levels were increased following FSO treatment. In contrast, expression levels of pro-inflammatory cytokines, such as IL-17A and IFN-γ, were elevated in aged muscle, which was reduced following FSO treatment. This finding suggests that FSO could increase Treg accumulation and function, decreasing inflammation in the muscle.
Tregs in the muscle exhibit enhanced expression of ST2, an IL-33 receptor (Burzyn et al., 2013). The binding of IL-33 to ST2 upregulates NF-kB, ERK, p38, and JNK kinases, facilitating cytokine release, cell proliferation, and Areg secretion (Kuswanto et al., 2016).
In aged muscles, the IL-33 signaling pathway is significantly downregulated by decreased IL-33 expression and a reduced number of IL-33 secreting cells (Kuswanto et al., 2016). However, IL-33 injection can attenuate the aging-induced decrease in muscle regeneration via Treg expansion (Kuswanto et al., 2016). Injection of IL-33 can increase the number of Tregs in peripheral tissues, such as adipose tissue or the intestine. Moreover, IL-33 reportedly leads to Treg expansion in muscles (Kuswanto et al., 2016). Mice with ST2 deficiency in Tregs were found to exhibit decreased Treg accumulation in the muscle when compared with wild-type mice, along with reduced muscle regeneration ability (Kuswanto et al., 2016). In the present study, expression levels of IL-33 and ST2 were reduced in aged muscles, which were enhanced by FSO treatment. Moreover, Areg expression was reduced in aged muscle, which was enhanced by FSO treatment.
Areg, a specific growth factor secreted by Tregs in the muscle, is well-known to directly affect satellite cells and promote muscle regeneration (Burzyn et al., 2013). Tregs are also involved in macrophage polarization toward M2 (Burzyn et al., 2013). M2 macrophages are directly involved in the differentiation of activated satellite cells, which generate myotubes and form myofibers for muscle regeneration post-injury (Tidball & Wehling-Henricks, 2007). In the present study, the expression of the M1 marker (CD86) was increased, while that of the M2 marker (CD206) was decreased in the aged muscle. FSO treatment decreased the expression of CD86; however, FSO increased the expression of CD206.
Adult satellite cells typically remain in a quiescent state and express PAX7 (Brack & Rando, 2012). However, satellite cells are activated by stress or injury and begin proliferating and migrating through the sarcolemma to fuse with multinucleated myofibers (Dumont et al., 2015). Following the initiation of proliferation, PAX7 expression decreases, whereas muscle-specific transcription factors MyoD and myogenin are increased (Dumont et al., 2015). Areg treatment upregulated the expression of PAX7, a myotube activation marker (Myf5), and a myotube differentiation marker (myogenin) (Jin et al., 2018). Herein, PAX7 expression in aged muscles was decreased; FSO treatment increased expression. Myogenesis markers, including Myf5, MyoD, and myogenin, were decreased in the aged muscle; FSO treatment increased these expression levels. Conversely, protein degradation markers such as atrogin-1 and MuRF-1 were increased in aged muscle, and FSO treatment decreased expression levels. Muscle mass, which was evaluated by muscle fiber CSA, muscle weight/body weight, and thickest diameter of the muscle, was reduced in aged mice, which was enhanced by FSO treatment. Muscle function, as evaluated by grip strength, was decreased in aged mice and increased following FSO treatment. FSO administration attenuated muscle mass and function by modulating satellite cell accumulation and function.
A reduced ability of satellite cells to repair damaged muscle fibers is considered one of the primary mechanisms underlying sarcopenia (Jang et al., 2011). Decreased satellite cell function and accumulation can be correlated with reduced regenerative potential (Collins et al., 2007). Our results revealed that FSO could attenuate aging-induced sarcopenia by increasing satellite cell accumulation and the myogenic ability of satellite cells.
In conclusion, the fermentation process increases muscle-enhancing biochemical substrates including GABA, lactate, BCAAs and FAAs. Furthermore, FSO enhanced autophagy in Tregs, increasing Treg accumulation and function in aged muscles. FSO increased Treg function, which, in turn, enhanced anti-inflammatory cytokines (IL-10 and TGF-β) and reduced pro-inflammatory cytokines (IL-17 and IFN-γ). In addition, FSO could lead to increased IL-33 and M2 polarization, which eventually elevates Areg, subsequently increasing satellite cell accumulation, as well as their myogenic function. Thus, FSO attenuated the age-associated decrease in muscle mass and function.