Introduction
The olive flounder, Paralichthys olivaceus, has an oval body shape resembling an olive. This fish occurs mainly in the benthos at depths of 10 to 200 m and is one of the most important marine fish species for commercial aquaculture in northeast Asia, including Korea and Japan (Lim & Hur, 2018). In 2014, approximately 42,000 tons of this species were farmed in Korea (Lim & Hur, 2018). Olive flounder culture and marketing procedures require grouping the fish by size by selecting individuals with similar growth patterns. The selection procedure includes numerous acute and chronic stressors to the fish, such as water level reduction in the rearing tank, size selection, capture, confinement, air exposure, and transport (Eslamloo et al., 2014). Olive flounder producers carry out these selection procedures without understanding how these acute and chronic stressors may affect the fish physiologically. Japanese croaker (Nibea japonica) is widely distributed along with East Asia, has a strong ability to adapt to adverse conditions, and is highly resistant to disease. In addition, Japanese croaker swimbladders are one of the by-products of the food processing industry (Chen et al., 2019).
The olive flounder and Japanese croaker have recently become important fish species in aquaculture in Korea and other parts of East Asia. The olive flounder is the most cultured fish among the farmed fish in Korea, and the Japanese croaker production has been increasing in recent years due to the rising water temperature (WT). Olive flounder and Japanese croaker farming and marketing procedures require grouping the fish by size by selecting individuals with similar growth patterns. The selection procedure includes numerous acute and chronic stressors to the fish, such as water level reduction in the rearing tank, size selection, capture, confinement, air exposure and transport (Hur et al., 2019).
WT, dissolved oxygen concentration (DO), and photoperiod are potent influences on feed consumption, metabolic rate and energy expenditure, and thus, on growth of poikilothermic vertebrates, including fish (Dutta, 1994; Elliott, 1982; Hur, 2018; Lee et al., 2014). Therefore, the effects of these environmental factors on fish growth and metabolism warrant thorough investigation.
It is well-known that stressed fishes secrete excessively catecholamine and corticosteroid hormone, altering their metabolism, growth, immune system, reproduction and osmoregulation (Hur et al., 2019). In fish rearing, the stress is divided into artificial factors; such as handling, confinement, transportation and medical treatment, and environmental factors; such as rearing density, water quality, WT and salinity. Therefore, fish may change its metabolism and physical rhythm depending on its environment. These stresses on fish induce the releases of catecholamine and cortisol in fish and these cause rapid metabolism of stored energy, harming health by biochemical effect and slow growth. Fish showed primary, secondary, and tertiary responses against the stress. The primary response showed rapid plasma catecholamine and corticosteroid (Hur, 2018). When this response to stressful conditions exceeded normal level, harmful secondary and tertiary responses occurred. Therefore, stress decreases energy metabolism, growth rate, and distracted reproduction. Significantly, the WT is an essential factor so that it controls physiological factors; reproduction, nutritional metabolism, growth, etc. Therefore, its rapid variation makes change and break physical condition and homeostasis of fish (Barton & Iwama, 1991; Hur, 2018; Lee et al., 2014; Shin et al., 2018). Therefore, this study was performed to get primary data for repeated acute change of WT.
One environmental stress factor, WT change, directly affects rearing fish’s metabolism. Barton & Iwama (1991) suggested that a rapid shift in WT resulted in in vivo metabolism and hematology changes. Rapid drop of WT such as cold-water mass, often occurring along the coast of eastern waters of Korea in summer, affects the growth and survival of farm fish (Chang et al., 2001). On the other hand, the increasing of in WT due to heated effluent water from power plant, and high WT during the summer season affects also the health of fish in culture farm. The cooling system in power plant makes water at about 7°C higher than natural seawater. This heated effluent water caused rapid change in WT at an adjacent coast. The rearing fish cannot quickly adapt to such temperature changes and the growth and survival of farm fish will be directly influenced by these changes (Chang et al., 2001). Hur (2018) examined effects of sudden change in WT on the stress responses of the cultured olive flounder. The secretion of cortisol, glucose, and lactic acid of olive flounder were significantly increased by WT. Hematological response and plasma osmolality of olive flounder were significantly increased by WT change. The respiration numbers per minute after WT increased were significantly higher than those before WT increased.
The present study determined the effects of WT changes on stress response and hematological factors of olive flounder and Japanese croaker. Such knowledge would help evaluate current olive flounder and Japanese croaker culture procedures and develop techniques to minimize stress during aquaculture.
Materials and Methods
Olive flounder (mean length 16.5 ± 0.2 cm, mean weight 40.4 ± 1.3 g, n = 20) and Japanese croaker (mean length 12.9 ± 0.2 cm, mean weight 21.8 ± 1.1 g, n = 20) used in this experiment were hatched in April 2019 at an aquaculture farm and subsequently transported to a mariculture facility at the Kunsan National University, Korea. During the acclimation and the following experimental period, the fish were fed commercial extruded pellet containing 56.0% crude protein and 12.0% crude lipid. Food was withheld from the fish for 24 hours before each experiment. Fish rearing (flow-through system) densities of each fish were 25.3 kg/m3 (80 flounders) and 6.5 kg/m3 (80 croakers), each 4 tanks. The seawater’s WT, salinity, and DO during the period ranged 22.7–23.2°C, 33–35 psu and 5.8–6.4 ppm, respectively.
Fig. 1 showed changes of WT designed in this experiment. WT increased from 23°C (control WT) to 26°C within 6 hours (0.5°C/hour) and then maintained at 26°C for 96 hours. WT decreased from 26°C to 23°C within 6 hours (0.5°C/hour), and was maintained at 23°C for 96 hours. WT increased from 23°C to 29°C within 6 hours (1°C/hour) and then maintained at 29°C for 96 hours. WT decreased from 29°C to 23°C within 6 hours (1°C/hour), and was maintained at 23°C for 96 hours. WT increased from 23°C to 32°C within 6 hours (1.5°C/hour) and then maintained at 32°C for 96 hours. WT decreased from 32°C to 23°C within 6 hours (1.5°C/hour), and was maintained at 23°C for 96 hours (Fig. 1).
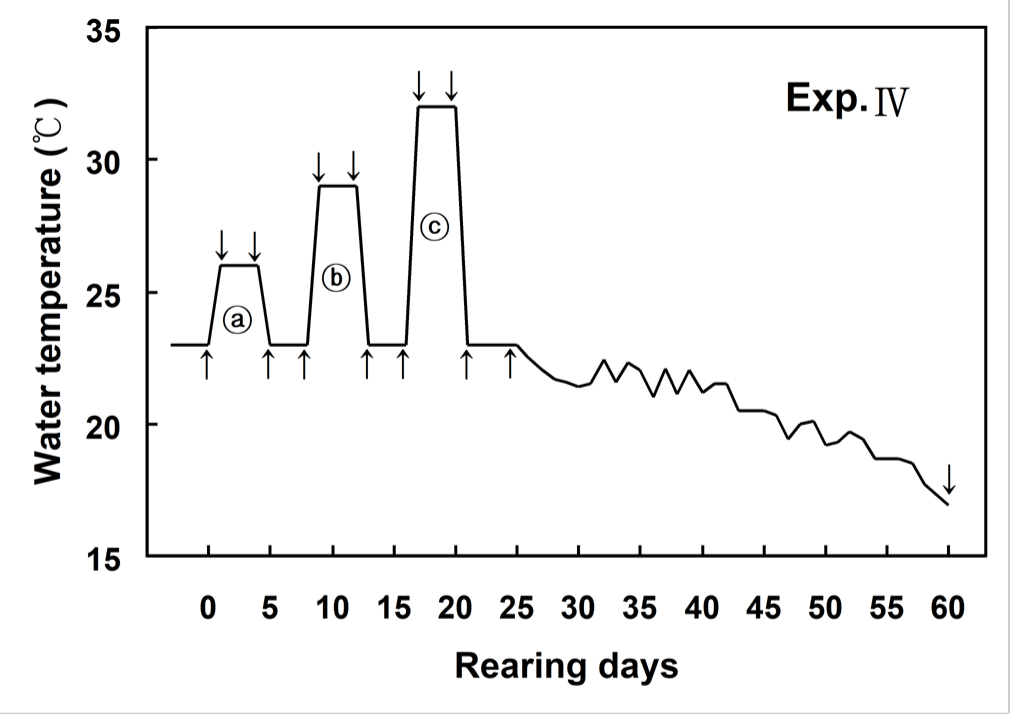
The blood of olive flounder and Japanese croaker were sampled from different tanks before and after the experiment. Blood samples were extracted from six olive flounder and eight Japanese croaker at 0 (before start of the experiment), 1, 5, 9, 13, 17, 21 and 60 days, respectively. Blood samples were collected from the caudal blood vessel complex using heparinized syringes within 1 min without anesthesia.
Hematocrit, red blood cells, and hemoglobin were analyzed immediately using an automatic blood analyzer (BC-2800VET, Mindray, Shenzhen, China). Blood samples were kept in 4-mL sodium heparin blood collection tubes (Becton Dickinson, Franklin, NJ, USA) and in 1.5-mL polypropylene microcentrifuge tubes held on ice for less than 5 min before centrifugation at 5,600×g for 5 min. Plasma was then collected and stored in a deep freeze (CLN-500 UW Nihon Freezer, Nihon Freezer, Tokyo , Japan) at –70°C until analysis. Plasma cortisol concentrations were determined in 50-µL samples using cortisol analyzer kits (Fuji, Tokyo, Japan) and cortisol analyzer (DRI-CHEM, Fuji). Glucose, lactic acid, aspartate aminotransferase (AST) and alanine aminotransferase (ALT) were analyzed using an automatic chemistry analyzer (DRI-CHEM NX 500i, Fuji). Osmolality was determined using a micro-osmometer (Fiske 210, Fiske, Norwood, MA, USA).
The experiment was performed in triplicate and results are reported as means ± SD (olive flounder n = 6, Japanese croaker n = 8) unless otherwise stated. One-way analysis of variance analyzed data with the SPSS statistical package (SPSS, Chicago, IL, USA). Means were separated by using Duncan’s multiple range test and were considered significantly different at p < 0.05.
Results
Hematological changes in fish in increasing WT are shown in Table 1. During the experimental period, the variations in the hematocrit of the non-stressed groups were 12.0 ± 0.1% to 19.0 ± 0.9%, 9.4 ± 0.0% to 15.3 ± 3.9%, and 14.2 ± 1.4% to 22.1 ± 0.5% in the olive flounder and Japanese croaker, respectively (Table 1). The changes in the hematocrit value of the olive flounder were temperature-dependent. When the WT was increased by 3°C in the stressed groups, the hematocrit value of the olive flounder increased significantly from 16.2 ± 1.9% (at the beginning of the experiment) to 17.2 ± 1.5% on day 1 and 19.4 ± 4.6% on day 5. However, the hematocrit value of the Japanese croaker decreased significantly from 21.7 ± 2.6% to 14.9 ± 3.5% on day 5. When the WT was increased by 6°C, the hematocrit value of the olive flounder and Japanese croaker increased significantly by day 9. When the WT was increased by 9°C, the hematocrit value of the Japanese croaker increased significantly by day 17. In contrast, the hematocrit values of the olive flounder did not differ significantly on day 17.
During the experimental period, the variations in the number of red blood cells in the non-stressed groups were 12.0 ± 0.1 to 19.0 ± 0.9 × 106 cells in the olive flounder and 14.2 ± 1.4 to 22.1 ± 0.5 × 106 cells in the Japanese croaker (Table 1). During the experimental period, the variations in the number of red blood cells in the stressed groups were 1.2 ± 0.3 to 3.3 ± 0.8 × 106 cells in the olive flounder and 1.4 ± 0.3 to 3.4 ± 0.8 × 106 cells in the Japanese croaker. The variations in the number of red blood cells were more significant in the Japanese croaker than in the olive flounder (Table 1). During the experimental period, the variations in the hemoglobin concentration in the non-stressed groups were 8.7 ± 0.3 to 15.4 ± 0.4 g/dL in the olive flounder and 6.9 ± 0.5 to 14.0 ± 0.3 g/dL in the Japanese croaker (Table 1). The variations in the hemoglobin concentration in the stressed groups were 5.5 ± 1.4–13.8 ± 2.8 g/dL in the olive flounder and 6.8 ± 1.1 to 13.2 ± 3.3 g/dL in the Japanese croaker during the experimental period. Thus, the variations in the hemoglobin concentration were more significant in the olive flounder than in the Japanese croaker (Table 1).
The cortisol concentration in the olive flounder increased from 5.1 ± 1.6 ng/mL (at the beginning of the experiment) to 5.1 ± 1.7 ng/mL, but did not differ significantly when the temperature was increased by 3°C in the stressed group (Fig. 2). The cortisol concentration in the olive flounder decreased significantly to 2.6 ± 1.1 ng/mL when the temperature was reduced by 3°C in the stressed group (p < 0.05). At 17 days, the cortisol concentration in the olive flounder was significantly increased to 76.9 ± 75.9 ng/mL when the temperature was increased by 9°C in the stressed group (p < 0.05), but was significantly reduced to 4.2 ± 1.2 ng/mL at 60 days (p < 0.05, Fig. 2). The glucose concentration in the olive flounder increased from 28.5 ± 0.7 mg/dL to 29.0 ± 1.4, 30.0 ± 1.4, and 31.0 ± 5.7 mg/dL when the WT was increased by 3°C, 6°C, and 9°C, respectively, in the stressed groups. The lactic acid concentration in the olive flounder was not significantly affected by changes in the WT in the stressed group (p > 0.05). However, as shown in Fig. 2, the cortisol concentration in the Japanese croaker increased significantly from 8.4 ± 1.2 ng/mL to 35.4 ± 3.2 ng/mL when the WT was increased by 3°C in the stressed group (p < 0.05). The cortisol concentration in the Japanese croaker decreased significantly to 4.3 ± 1.8 and 2.6 ± 2.5 ng/mL when the WT was increased by 6°C and 9°C, respectively, in the stressed group (p < 0.05). However, the cortisol concentration in the Japanese croaker increased significantly to 91.4 ± 28.9 ng/mL at 60 days (p < 0.05). In the control group of the Japanese croaker, cortisol increased from 6.8 ± 2.4 ng/mL to 106.5 ± 35.6 ng/mL over 60 days (p < 0.05). The glucose concentration in the Japanese croaker increased significantly from 41.0 ± 2.8 mg/dL to 61.5 ± 0.7, 62.0 ± 9.9, and 80.0 ± 17.0 mg/dL when the WT was increased by 3°C, 6°C, and 9°C, respectively, in the stressed groups (p < 0.05). At 60 days, the glucose concentration in the Japanese croaker decreased to 41.5 ± 2.1 ng/mL in the stressed group (p < 0.05). The lactic acid concentration in the Japanese croaker increased to 2.5 ± 1.3 mmol/L by day 5 (p < 0.05), but was not significantly altered at 9–60 days after the WT was changed in the stressed groups (p > 0.05). The cortisol concentration in the olive flounder did not differ significantly between WTs of 23°C and 26°C, but the cortisol concentration was significantly higher at WTs of 29°C and 32°C than at WTs of 23°C and 26°C. The cortisol concentration in the olive flounder increased when the WT was repeatedly altered. However, the cortisol concentration in the Japanese croaker decreased under these conditions and was highest on day 60. Therefore, the Japanese croaker has a greater tolerance of high WT than the olive flounder.
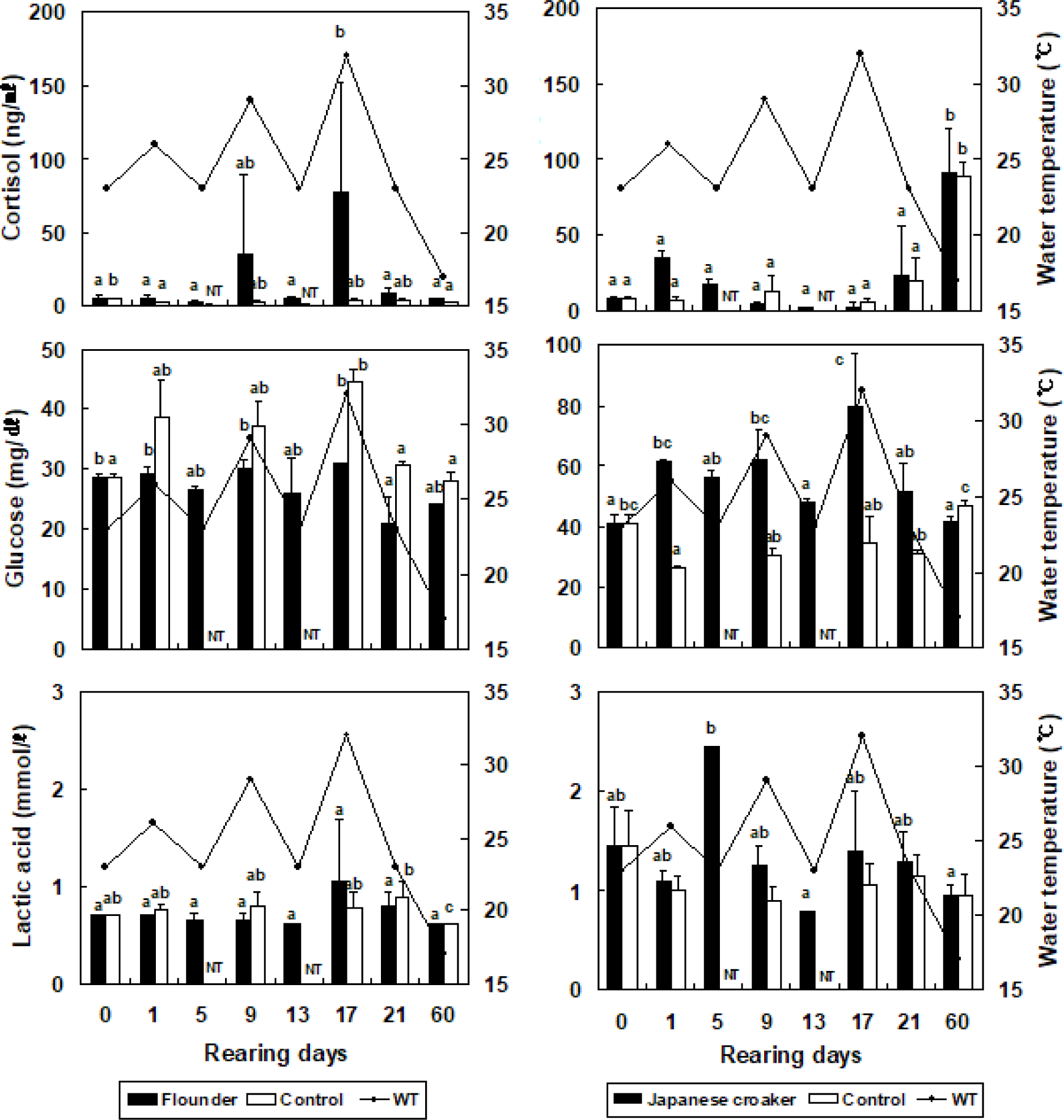
During the experimental period, the variations in osmolality in the non-stressed groups were 421.0 ± 29.7 to 444.5 ± 6.4 mOsm/kg in the olive flounder and 326.5 ± 0.7 to 345.5 ± 2.1 mOsm/kg in the Japanese croaker (Table 2). The variations in osmolality in the stressed groups were 386.5 ± 5.0 to 447.0 ± 5.7 mOsm/kg for the olive flounder and 324.0 ± 2.6 to 339.5 ± 5.0 mOsm/kg for the Japanese croaker. Thus, the variations in osmolality were smaller in the Japanese croaker than in the olive flounder (Table 2). The variations in AST in the non-stressed groups were 20.4 ± 0.1 to 24.3 ± 2.1 IU/L in olive flounder and 15.2 ± 12.9 to 21.7 ± 1.6 IU/L in the Japanese croaker during the experimental period (Table 2). The variations in AST in stressed groups were 23.9 ± 0.2 to 44.3 ± 2.8 IU/L in the olive flounder and 3.0 ± 0.8 to 23.3 ± 4.9 IU/L in the Japanese croaker. Thus, the variations in AST were more significant in the olive flounder than in the Japanese croaker (Table 2). The variations in ALT in the non-stressed groups were 21.1 ± 1.0 to 23.9 ± 0.2 IU/L in the olive flounder and 9.8 ± 1.9 to 12.1 ± 0.4 IU/L in the Japanese croaker (Table 2). During the experimental period, the variations in ALT in the stressed groups were 18.3 ± 0.2 to 32.7 ± 0.4 IU/L in the olive flounder and 1.4 ± 0.3 to 9.8 ± 1.9 IU/L in the Japanese croaker. Thus, the variations in ALT were lower in the Japanese croaker than in the olive flounder (Table 2).
Discussion
Many recent studies have investigated the stress response, reproductive capacity, and metabolic physiology of fish mediated by the hypothalamic-pituitary–interrenal axis (Hur, 2018; Lee et al., 2014; Shin et al., 2018). Barton & Iwama (1991) suggested that fish cope adequately with stresses arising from changes in their external environments but that stress above a certain threshold reduces their physiological activity and harms their health. Fish experiencing frequent stress events find it difficult to maintain homeostasis and require energy to overcome the stress. Because this energy is diverted from normal body growth and the maintenance of vital processes, growth retardation occurs and the mortality rate increases (Barton & Iwama, 1991; Hur, 2018).
The trends in cortisol, glucose and lactic acid concentrations observed in this study represent generalized stress reactions. The glucose concentrations increased simultaneously with the increases in cortisol concentrations. These increases in cortisol and glucose concentrations seemed to correlate with the increase in WT changes-induced stress. This finding is consistent with previous findings that elevated stress levels are accompanied by increases in plasma cortisol and glucose concentrations (Chang et al., 2001; Cho et al., 2015; Hur, 2018; Hur et al., 2019; Jaxion-Harm & Ladich, 2014; Lim & Hur, 2018).
Some results in our experiment were consistent with those of Jaxion-Harm & Ladich (2014) and Cho et al. (2015), but others were not. The hemoglobin concentrations of the olive flounder decreased with increasing WT, whereas those of the Japanese croaker increased with increasing WT. The cortisol concentrations of the olive flounder increased with increasing WT, whereas those of the Japanese croaker increased with decreasing WT. However, the cortisol and glucose concentrations of the olive flounder did not change significantly between the beginning and the end of this experiment. It is possible to quantify the adverse effects of stress by directly measuring endocrine responses, such as cortisol production (Jaxion-Harm & Ladich, 2014; Tanck et al., 2000; Wysocki et al., 2006). Under stressful conditions, the hypothalamus releases corticotropin factor, which stimulates the anterior pituitary gland to produce adrenocorticotrophic hormone, thereby activating the release of cortisol from the interrenal tissue into the bloodstream. The primary function of cortisol is to induce physiological changes that help fish either protect themselves from or adapt to the stressor. Physiologically, cortisol increases metabolic, heart, and ventilation rates. However, continuously high cortisol concentrations can become maladaptive because they impair the immune system, which may ultimately affect the fish’s health (Jaxion-Harm & Ladich, 2014).
In both species examined in this study, the glucose concentrations increased with increasing WT. Catecholamines may rapidly elevate glucose concentrations, but further increases are usually caused by corticosteroids, which facilitate gluconeogenesis (Barton & Iwama, 1991; Hur, 2018; Lim & Hur, 2018). This response rapidly provides muscle tissues with large amounts of glucose to support the organism during acute stress. Gluconeogenesis activation toward enzyme increases, and the secretion increases when the fish secrete cortisol in response to the stressor (Barton & Iwama, 1991; Lim & Hur, 2018). Barton & Iwama (1991) suggested that this increase results from a second reaction to the first reaction (hormone response) to stress. Balta et al. (2017) noted that the plasma glucose concentration was more significant in the experimental group at 1 hour after acute WT stress than in the control group. The highest glucose concentration in the stressed group (up to 61.8 ± 2.55 mg/dL) was reached within 1 hour and started to decline at 24 hours, decreasing to the concentration in the control group (25.4 ± 3.86 mg/dL) at 72 hours. Biswas et al. (2006) reported that acute stress caused an increase in the glucose concentration in the red sea bream (Pagrus major), which began to decline 24 hours later and returned to the normal concentration after 72 hours. Kubilay & Uluköy (2002) reported increasing amounts of serum glucose in the rainbow trout (Oncorhynchus mykiss) after acute stress (transport, screening, and compression). In the present study, the cortisol and glucose concentrations of the stressed olive flounder did not return to normal as WT increased, and those of the Japanese croaker increased after 21 days (decreasing WT). This suggests that the stress induced by the change in WT was not relieved within 12 hours. The change in WT resulted in tension-induced activity in the fish, elevating both cortisol and glucose concentrations. However, the change in WT did not result in the generation of lactic acid. This suggests that the energy used by the fish was not supported by anaerobic metabolism. Lim & Hur (2018) suggested that flatfish store lactic acid in muscle tissues rather than discharging it into the blood, even under low-oxygen conditions. We suggest that the lactic acid concentrations did not change in the fish in the present study because lactic acid was not discharged from the muscle into the blood after the change in WT.
Many studies have shown that the handling, confinement, vaccination, and grading of fish and acute or chronic fluctuations in WT cause a rapid and temporary increase in plasma cortisol concentrations, which causes a primary stress response in the fish (Balta et al., 2017). Secondary physiological responses lead to increased glucose concentrations as an energy source and in lysozyme, which is involved in the innate defense mechanism. Cortisol is the most critical glucocorticoid secreted by the interrenal kidney tissue in bony fish. Many researchers have reported that plasma cortisol concentrations increase in fish exposed to stress. In most fish, cortisol concentrations increase rapidly after stress and return to the initial concentrations within 6–48 hours (Balta et al., 2017). Therefore, increasing WT likely imposed a more stressful environment on the olive flounder than on the Japanese croaker, and decreasing WT imposed a more stressful environment on the Japanese croaker than on the olive flounder. In the present study, the plasma lactic acid concentrations in the olive flounder and the Japanese croaker were not significantly different after the change in WT. This result is inconsistent with reports showing that stress stimulates lactic acid generation in fish (Jiang et al., 2017; López-Patiño et al., 2014; Morales et al., 2005). No significant differences were observed in plasma lactic acid concentrations when WT was increased or reduced, suggesting that changes in WT do not result in significant changes in plasma lactate concentrations in the olive flounder or Japanese croaker.
In this study, the cortisol and glucose concentrations of the olive flounder tended to increase with increasing WT, whereas those of the Japanese croaker decreased with increasing WT. Therefore, the metabolic activity of the olive flounder increased in direct proportion to the increase in WT, whereas the metabolic activity of the Japanese croaker showed an opposite response. Furthermore, the cortisol and glucose concentrations of the olive flounder increased in proportion to the increase in WT, which is consistent with the principle of the thermal quotient (Q10 value) as a metabolic index. Our results should help evaluate the current culture procedures used for the olive flounder and Japanese croaker and develop techniques to minimize stress during aquaculture.