Introduction
Aquaculture has experienced rapid growth in the past two decades. World aquaculture production increased from 35 million tons in 1997 to 114.5 million tons in 2018 (FAO, 2020). This pattern of growth has also occurred in Vietnam but faster with a nearly 100-fold increase in production from 492 thousand tons in 1997 to 4.8 million tons in 2021, with an export value of 8.89 billion USD. Production of farmed shrimp in 2021 was estimated at 930 tons of which 632 tons was whiteleg shrimp (Penaeus vannamei), 268 tons was tiger shrimp (Penaeus monodon), and the remainder was other shrimp species (TCTS, 2021). Due to the rapid growth of the aquaculture industry in Vietnam there have been issues with environmental degradation and disease outbreaks. One of the diseases causing severe losses to the shrimp industry is acute hepatopancreatic necrosis disease (AHPND), caused by the gram-negative bacterium Vibrio parahaemolyticus. It is estimated that losses from AHPND in the Mekong Delta region between 2011 and 2015 were approximately 26 million USD for whiteleg shrimp and 11 million USD for black tiger shrimp (FAO, 2018). There is currently no effective control measure for this disease as antibiotics are not effective. The industry therefore needs to find alternative measures to prevent and control this pathogen.
Nanobubble technology produces small gas bubbles with a diameter of less than 200 nm (Agrawal et al., 2011; Chaplin, 2021) which can exist in the water column for weeks (Azevedo et al., 2016; Parmar & Majumder, 2013). This phenomenon is associated with the physical characteristic of these small bubbles (Weijs & Lohse, 2013) and high zeta potential value which keeps them from aggregating into larger bubbles (Kirby, 2010; Sjogreen et al., 2018). Nanobubble technology has a lot of promising effective application in aquaculture because of its ability to increase and maintain oxygen content in water and control bacteria, a possible measure to reduce the use of antibiotics.
Oxygen nanobubbles optimize the dissolved oxygen in aquatic systems (Galang et al., 2019). High levels of oxygen may speed up metabolism, promote higher food intake, and increase growth of farmed shrimp (Ebina et al., 2013). Ozone nanobubble has recently been shown to effectively disinfect water in laboratory experiments and small mesocosm experiments (Imaizumi et al., 2018; Jhunkeaw et al., 2021; Nghia et al., 2021; Seki et al., 2017). The fundamental disinfection properties of ozone nanobubbles are that they attract negatively charged particles and create oxygen free radicals which disrupt the permeability of cell membranes (Gurung et al., 2016; Ikehata & Li, 2018; Temesgen et al., 2017). In water, ozone is converted to oxygen and therefore it increases dissolved oxygen levels while disinfecting water (Nano Bubble Technologies, 2020). In marine recirculating aquaculture system moderate ozonation seems to promote biological nitrification. This is likely mediated by the increase in oxygen promoting nitrogen converting bacterial populations. The limiting factor for the use of ozone in salt water is the toxicity to the fish (Schroeder et al., 2015). Effective ozone treatment depends on concentration, exposure time, pathogen load and organic matter content. The higher the ozone concentration, the higher the risk to famers and farmed species (Gonçalves & Gagnon, 2011).
Although nanobubble can increase and maintain oxygen in water, gas overdose can cause hyperoxia. The effect of air supersaturation on aquatic animals has documented in many studies. Hyperoxia caused a 67% increase in total interlamellar cell mass (Tzaneva et al., 2011). Boyd & Fast (1992) reported that fish and shrimp mortality increased when oxygen exceeded 20 mg/L and or the saturation rate exceeded 300%. This condition can result in gas bubble disease (gas bubble trauma), which has been described in a wide variety of fishes and invertebrates. Weitkamp & Katz (1980) reported that bubbles were observed in the gills of dying fish as well as between fin rays and under scales when dissolved oxygen levels are over 300% saturation and numerous marine fish died when dissolved oxygen concentrations were above 250% of saturation in Galveston Bay. Anecdotal reports from shrimp industries in Asia suggests oxygen nanobubble technology is effective at increasing dissolved oxygen in pond shrimp culture. However, despite some knowledge of the physical and chemical properties of nanobubbles, much remains unknown about their effect on aquatic animals (Atkinson et al., 2019).
In this study, we investigated the effects of nanobubbles of oxygen and ozone gases on gill morphology, weight gain and mortality of Pacific white shrimp (P. vannamei) as well as V. parahaemolyticus load and water quality of shrimp culture tanks under laboratory conditions.
Materials and Methods
Experiments were carried out from May to September 2020 in a laboratory at the Centre for Environment and Disease Monitoring in Aquaculture (CEDMA), Research Institute for Aquaculture No. 1, Bac Ninh, Vietnam (RIA1). Trials were conducted in 140 L cylindrical composite tanks with 100 L of 15‰ saline water, which is optimal for V. parahaemolyticus (WHO & FAO, 2011). Saline water was prepared by adding 1.5 kg Blue Treasures Sea salt (Qingdao Sea-Salt Aquarium Technology, Qingdao, China) to 98.5 L fresh water.
A nanobubble generator model aQua+75MO (AquaPro Solutions, Singapore, Singapore) with a 1 HP power (0.75 kW) motor and a water flow output rate of 1,000 L/h was used to generate nanobubbles. The machine produced nanobubbles with an average size of 168.9 ± 73.8 nm, and a concentration of 1.04 × 109 ± 2.6 × 108 particles/mL (AquaPro Solutions, 2019). To create oxygen nanobubbles, we used an oxygen concentrator (model Yuwell 7F-10, Yuwell, Danyang, China; 10 L/min max flowrate, ≥ 95.5% oxygen concentration, flow rate at 4 L/min) provided oxygen for nanobubble generator. To create ozone nanobubbles we used the above mentioned oxygen concentrator provided oxygen to ozone generator (model OM-Z10, OzoneMaxx, Hanoi, Vietnam; output flow rate of 15 L/min, produce 10 grams of ozone per hour) to produce ozone, and using the ozone for nanobubble generator as described by Nghia et al. (2021).
Two separate experiments were designed to investigate the effects of oxygen nanobubbles and ozone nanobubbles on shrimp’s gill morphology, weight gain, and mortality. These experiments were also designed to study the effects of the gases nanobubbles to V. parahaemolyticus concentration in water, and physicochemical parameters of water in the experiment tanks.
Experiment 1 (without V. parahaemolyticus) consisted of five treatments: oxygen macrobubble (O2), ozone macrobubble (O3), oxygen nanobubbles (O2-NB), ozone nanobubbles (O3-NB), and negative control which was given air macrobubble (NCTRL). Each group was replicated three times. The O2 and O2-NB tanks were treated for 4 minutes once per day, while O3 and O3-NB tanks were treated for 2 minutes once per day. Optimal treatment times were set based on previous research results (Nghia et al., 2021) and a pilot trial prior to this experiment. There were no bacteria added to the systems in Experiment 1 (only in Experiment 2), but we monitored bacterial count in the water to determine if different gases could prevent bacterial proliferation and improve water quality. The treatments were continued for 21 days.
Experiment 2 (with V. parahaemolyticus) consisted of six treatments: O2, O3, O2-NB, O3-NB, NCTRL no V. parahaemolyticus exposure, only air macrobubble, and positive control (PCTRL) exposed to V. parahaemolyticus and air macrobubble. Each treatment was replicated three times. The O2, O2-NB, O3-NB tanks were treated for 10 minutes once per day, while the O3 tanks were treated for 2 minutes once per day. Except for the NCTRL, all tanks in Experiment 2 were inoculated with V. parahaemolyticus at a concentration 106 CFU/mL. The reason we set 10 minutes treatments for all treatments except the O3 instead of the shorter exposure used in Experiment 1 because we needed more time to disinfect against the bacteria. O3 macrobubble treatment were set 2 minutes because macrobubbles has more effect to shrimp than nanobubbles, and a longer duration would have resulted in mortality of the shrimp (data not shown). Every 48 hours, 50 mL of Nutrient Broth culture medium was added to each tank to stimulate and maintain the optimum condition for bacteria growth. The treatments were continued for 21 days.
All tanks in both experiments stocked with 20 shrimp (40–45 days post-larva) from a local farm and supplied with an aquarium air-stone operated continuously throughout the trials. All animals were acclimated in a composite tank (100 L) at 15‰ salinity for 1 week prior to the study. Shrimp were fed 3 times a day for a total of 3% of their body weight and examined daily. Waste at the bottom of the tanks were collected daily, water was added to the tanks to compensate for water lost during cleaning. Moribund animals (i.e., shrimps that were weak, had no reaction, had no food in their gut, and sank to the bottom of the tank) were collected for sampling immediately. Water quality was monitored daily for 21 days.
Shrimp gill samples were prepared for histology following Lightner (1996). Sections (5 µm thickness) were stained with hematoxylin-Mayer and eosin. Preparations were observed under microscope (Nikon E200 LED, China) at 400× magnification. The degree of gill pathology was graded into 5 main levels: normal filament cells (L0), filament cells adhesion (L1), cell’s nucleus adhesion (L2), gill arch atrophy (L3), and gill arch caseation (L4). Each major level was further divided into 4 sub-levels (score) (e.g., L0.1 to L0.4) to indicate the severity of the gill damage. The number of analyzed samples (shrimp) from each treatment depended on the number of moribund caught shrimp and remaining live shrimp at the end of experiment. To obtain an overall score for each gill sample we multiplied the main level (e.g., 1–5) by the sub-level (e.g., 1–4) and these numbers were summed up for each shrimp, the calculation is expressed by the following formula:
Where x is the main level and y is sub-level.
These scores were then recoded to normal (total < 2) and then subsequently categorized into four groups based on quartiles of the scored data, i.e., light, medium, severe, and very severe pathology with approximately equal sample size. In order to have the same scale in both trials, we based the selection of cut-off points on trial 1 for both trials.
Before starting the experiment, shrimp were weighed individually using an analytical balance (PA214, Ohaus, Parsippany, NJ, USA) after removing water from the shrimp with a soft tissue paper. At the end of the experiment, live shrimp in the experimental tanks were weighed as described above. We calculated the average weight of the shrimp in each tank at the start and at the end of the study. We compared the change in average weight across tanks in different treatment groups.
The number of dead shrimps was recorded according to the experimental tank. Shrimp activity and status were checked two times a day during the experiments. Shrimp that sank to the bottom of the tank were collected immediately, euthanized, and fixed with Davison solution for histopathology analysis. A bacterial culture of the hepatopancreas on thiosulfate citrate bile sucrose agar (TCBS) media was done to test for V. parahaemolyticus to determine if this was the cause of death prior to fixation.
The AHPND strain of V. parahaemolyticus was collected from the bacteria storage bank in CEDMA, RIA1 and was prepared as follows: 1) V. parahaemolyticus bacteria were thawed from ultra-low temperature freezer –80°C and defrosted at room temperature; 2) the bacteria were cultured in selective medium (TCBS) in an incubator at 29°C. After 18–24 hours, single colonies were put into sterilized Erlenmeyer flask containing nutrient broth with 2% NaCl medium and shaken at 29°C for 18 hours; and 3) the bacterial concentration was optically estimated at OD600 nm with an Eppendorf Bio Spectrometer (Eppendorf, Hamburg, Germany) and enumerated by dilution and plating. Bacteria was introduced into experiment tank at the concentration 106 colony forming units per mL of water (CFU/mL).
Bacterial counts were measured at 0, 6, 12, 24 hours at the beginning of the experiments and thereafter once a day. The tank’s water was stirred before sampling. Bacterial concentration in water was quantified following Buller (2004). Collected water samples from experimental tanks were diluted 10-fold with 2% sodium chloride solution. A volume of 100 µL of dilution solution was inoculated on a TCBS agar plate and a glass was used to evenly spread the bacteria on the agar surface. Cultured TCBS agar plates were incubated in the incubator at 29.0°C. After 24 hours, colonies were counted, and the bacterial concentration was calculated using the following formula:
Where: X is bacterial concentration in 1 mL sample (CFU/mL), A is the number of colonies growing on agar plates, V is the volume of water introduced into the culture (for example, if 100 µL is added, V = 0,1), K is the dilution factor (for example, at a concentration of 10-1, K = 10). The minimal detection limit in our lab was 14 CFU/mL.
The hepatopancreas of each shrimp was dissected and DNA was extracted as described in MOSTE (2019). AP3 primer pairs with sequences: 5’-ATGAGTAACAATATAAAAACATGAAAC-3’ and 3’ GTGGTAATAGATTGTACAGAA-5’ was used to test for the presence of V. parahaemolyticus by PCR using gel electrophoreses to visualize results.
Water quality parameters including temperature, pH, oxygen (DO), alkalinity (Alk), oxidation reduction potential (ORP), nitrite (NO2), hydrogen sulfide (H2S), ammonia (NH3), ammonium (NH4+) were measured at 0, 6, 12, 24 hours, and thereafter once a day. Tank water was stirred before sampling. Temperature, DO, pH, ORP were measured by a Pro1020 Dissolved Oxygen and pH or ORP Instrument (YSI, Yellow Springs, OH, USA). Alk, NO2, NO3, H2S, NH3, NH4 were analyzed by the SMEWW 2302B: 2011, SMEWW 4500-NO2 B: 2011, 4500-NO3 B: 2011, SMEWW 4500-S2- D: 2011, SMEWW 4500-NH3 F: 2011 method, respectively (Baird & Bridgewater, 2017).
Gill scores were analyzed using ordinal logistic regression to compare categories (normal, light, medium, severe, and very severe changes) among treatments and to estimate probabilities for occurrence of these categories. Change in weight of shrimp was compared between treatments. An interaction between the two factors (treatment and time) was used in the linear regression. Total mortality at the end of the experiments was compared among treatments using a logistic regression model. In Experiment 2, survival curves were compared among treatments using the Cox proportional hazards model. We also depicted mortality curves using Kaplan-Meier failure function.
Log transformed bacterial counts (x) Log10(x + 1), and the water quality parameters were compared between treatments and over time using linear regression. We used an interaction term between time and treatment in the model to control for the repeated measures. For bacteria counts, the negative control was excluded from the analysis. Model assumptions were assessed using residual plots.
Analyses were carried out using XLSTAT statistical and data analysis solution (XLSTAT, 2020). The p-values < 0.05 were considered significant.
Results
In Experiment 1, all shrimp from the control group scored as normal, i.e., no gill change observed. The highest gill damage level L4.2 occurred in 18 shrimp in the O3 treatment, 7 shrimp in the O2 treatment, and 2 shrimp in O2-NB treatment. No L4.2 gill scores were observed in the O3-NB treatment (Table 1). The relative occurrence of the various composite gill change scores did not differ significantly between O2 and O2-NB treatment and had considerably fewer animals with severe gill damage than the O3 treatment (p < 0.001). Differences among the O3 treatment and the two O2 treatments were not significant (Fig. 1).
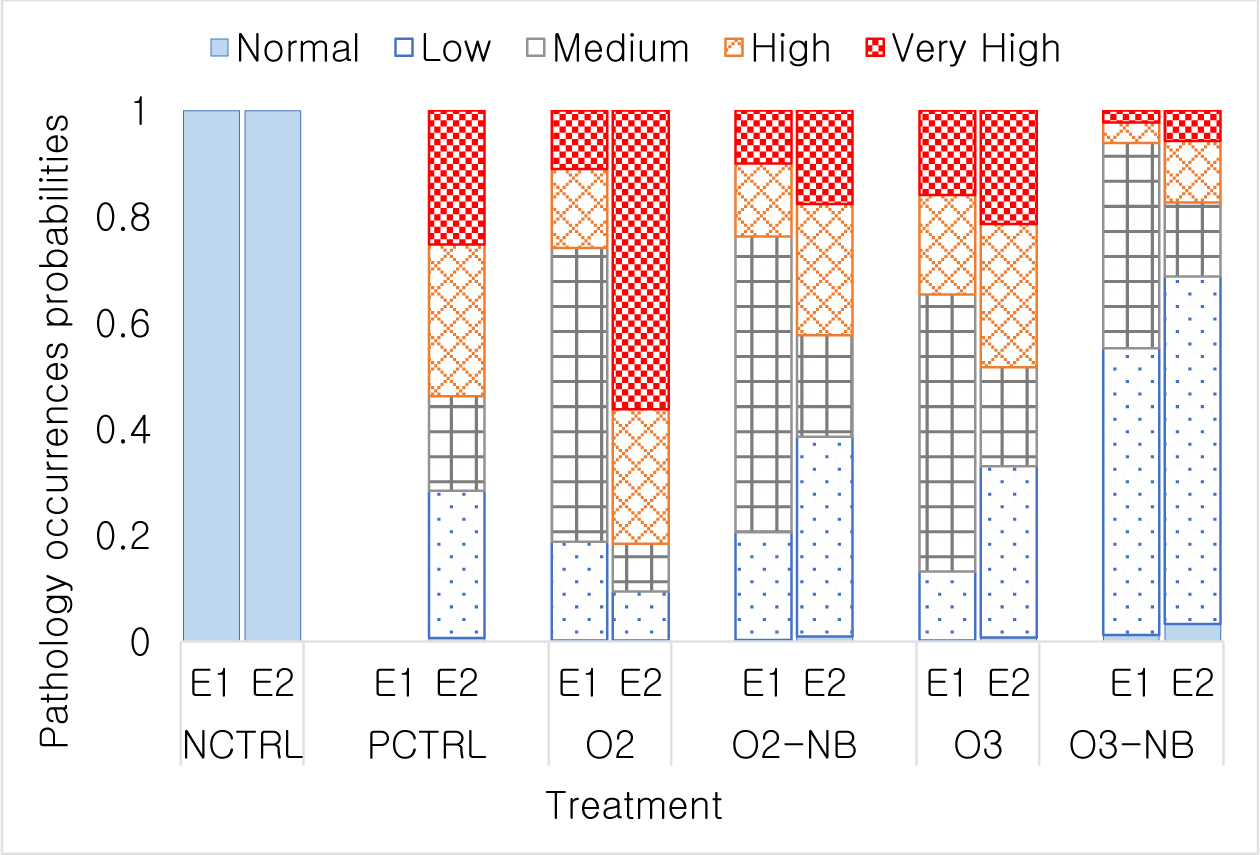
In Experiment 2, all treatments except the negative control had gill damage. There was a few shrimp (i.e., one in the positive control and four in the O2 treatment) had very high gill damage scores of (Table 1). All shrimp from the negative control and a few shrimp from the other treatments had normal scores. The two O2 treatments differed significantly (p < 0.001) from the O2-NB which had fewer animals with lower damage scores compared to the O2 treatment. The two O3 treatments also differed significantly (p < 0.01) in which O3-NB groups had only a few shrimp with very high gill damage scores. Relative occurrences of different gill scores were not different between the positive control, O2-NB, and O3 treatments (Fig. 1).
In Experiment 1, the size of shrimp did not vary significantly among treatments at the start of the experiment. The average weight gain in the control group at the end of the study was 0.7871 ± 0.044 g. The weight gain in the O3 treatment group was significantly lower than in the other four groups (p < 0.05). Differences in weight gain among the other four treatments did not differ significantly.
In Experiment 2, there were significant differences among the groups at the start of the experiment; the ozone group had lower mean weight than the other groups (p = 0.007). The average weight gain in the negative control group was 0.5977 ± 0.06 g and differences among treatments were not significant (Fig. 2).
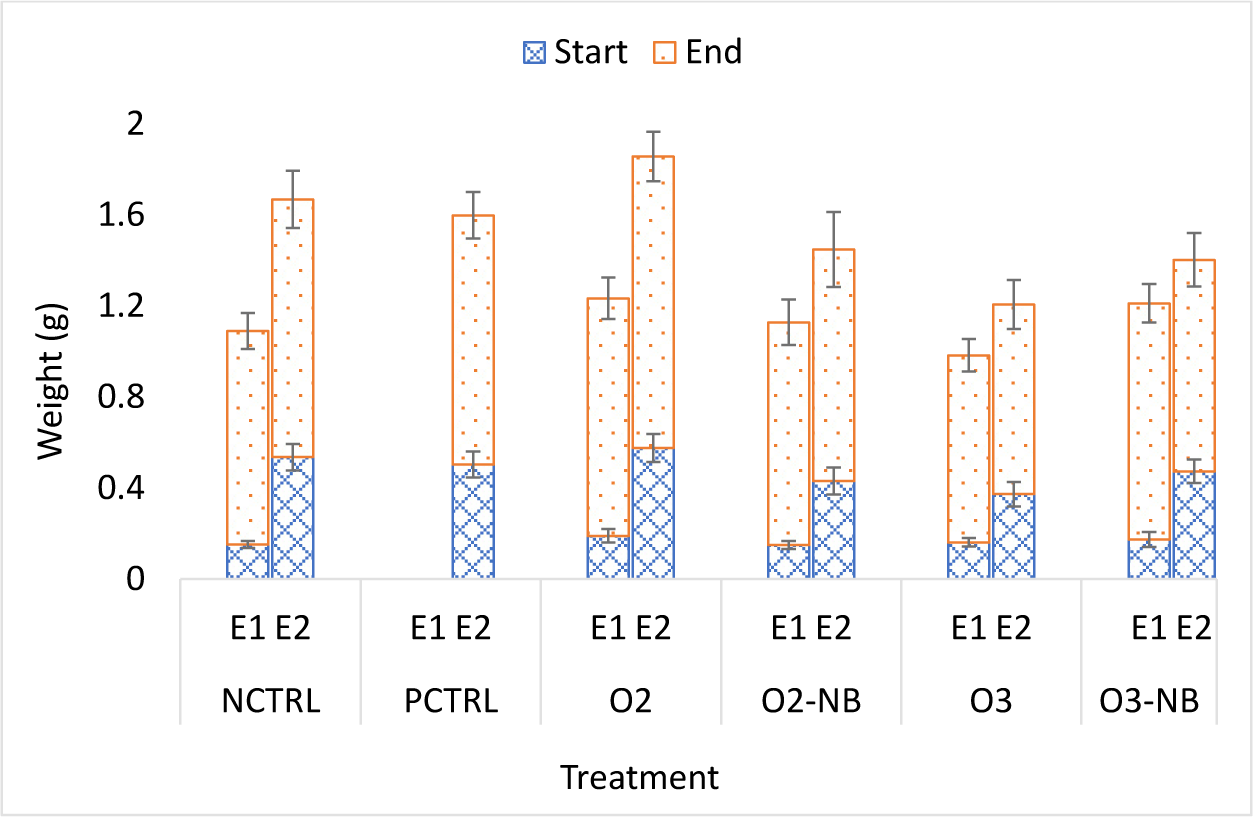
In Experiment 1, the odds of shrimp dying in the O3 treatment group was 4.67 times higher than in the control treatment (p < 0.05), while differences among the other treatments were not significant. There was not a significant difference in the odds of shrimp dying in the O2-NB group or in the O3-NB groups compared to shrimp in the control group (odds ratio [OR] = 1.27, p = 0.73; OR=1.85, p = 0.35).
In Experiment 2, the mortality rate in the negative control was 0.05 (5% of shrimps had died). All treatments had a higher mortality at the end of the study than the negative control. The odds of dying in the positive control was 28.5 times that of the negative control (p < 0.001). Similar mortality rates 62.4 (p < 0.001), 32.8 (p < 0.001) and 19 (p < 0.001) were detected in the O2 treatment, O2-NB treatment and the O3 treatment, respectively. The odds of dying in the O3-NB treatment was only 3.35 times that of the negative control group and this was not statistically significant (p = 0.081). Differences among PCTRL, O2 and O2-NB treatments were not statistically significant. Mortality was higher (p < 0.001) in the O3 treatment than in the O3-NB treatment (Fig. 3). Mortality curves differed significantly among treatments (p < 0.001). Mortality occurred during the first few days of the experiment, which was low after then.
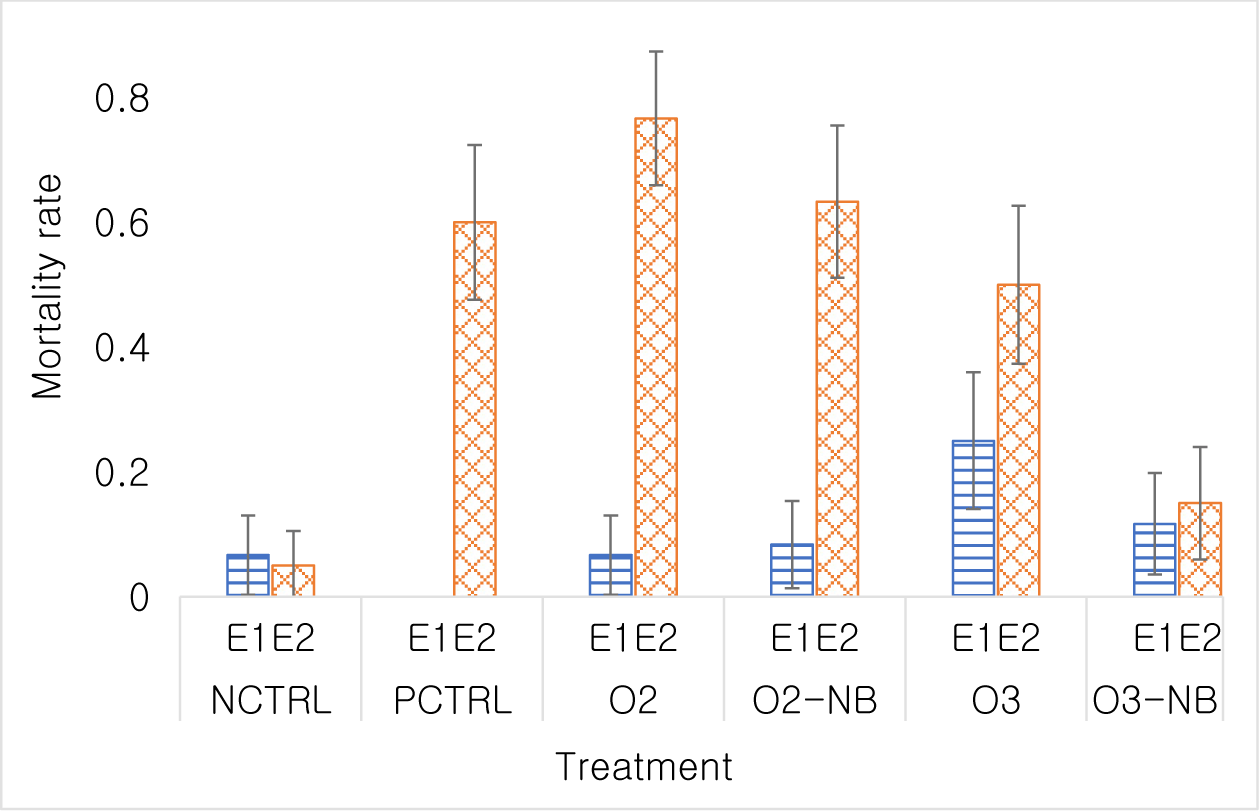
Changes in bacteria counts [as Log10(count + 1)] over time was modelled. Bacteria counts declined in a curvilinear manner with Log10(time in hours) and therefore we used polynomial regression to analyze the differences in patterns. Bacteria counts declined quickly in all tanks but particularly so in the two O3 treatment groups (Fig. 4). No bacteria were observed in the negative control. Bacterial counts at important milestones such as 6, 120, 240, and 480 hours of the experiment were compared using marginal means. By 6 hours post treatment bacteria counts were markedly lower in the two ozone treatments than in the positive control and the two oxygen treatments (p < 0.001). The same pattern was also observed at the other time points although bacteria density had gone down in all treatments.
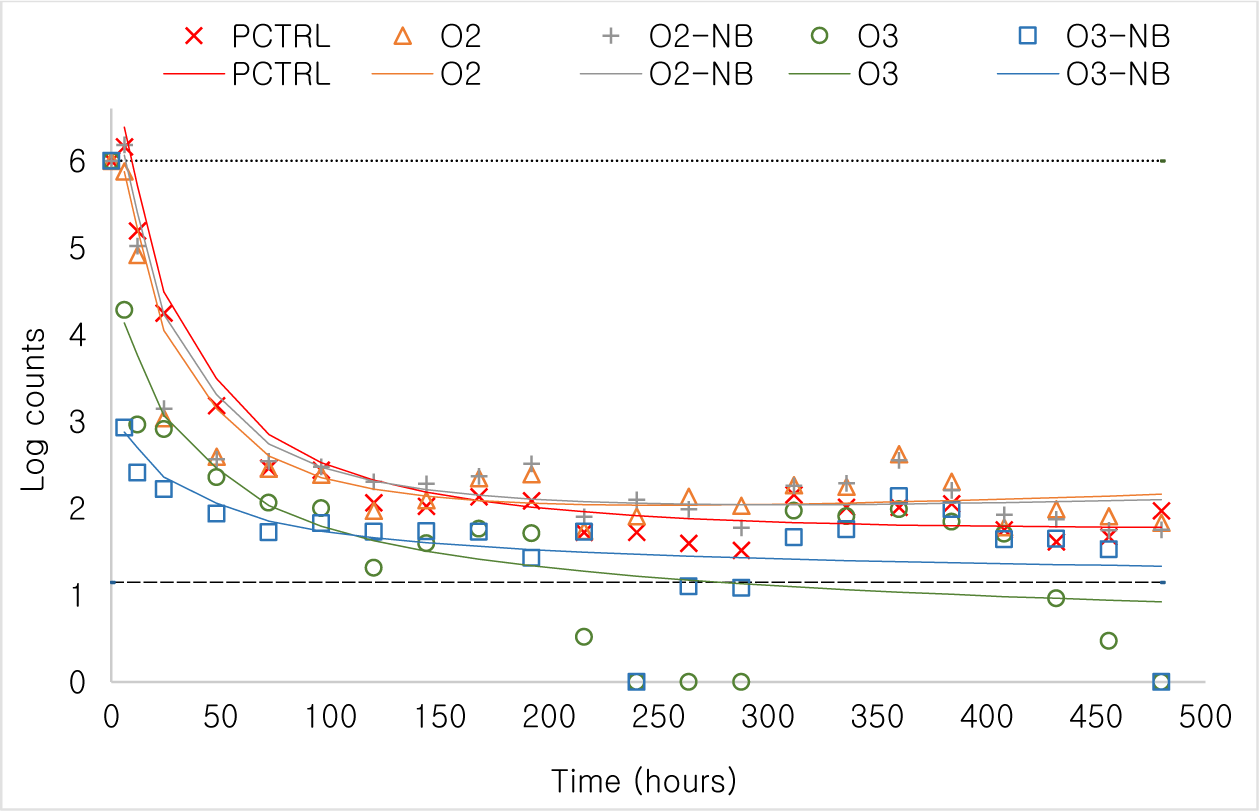
Additional, PCR test of dead and live shrimp suggest V. parahaemolyticus was found in 29 of 38 shrimp (76.3%) in the positive control. The odds of death associated with V. parahaemolyticus (ratio of positive and negative probability) was 0.5862 (n = 26), 0.6466 (n = 37), 0.3103 (n = 22) and 0.1194 (n = 18) in the O2, O2-NB, O3 and O3-NB treatments, respectively.
Temperature and pH in both experiments changed in a similar pattern for all treatments. Temperature in the NB treatment groups was significantly higher than the other treatments (p < 0.001). The pH level in the O2 treatments was significantly higher than in the O3 treatments (p < 0.001). In both experiments, ORP values of the O3 treatments were significantly higher than in other treatment groups (p < 0.001); and DO content of the O3 and O2 treatment tanks were significantly higher than the NCTRL and PCTRL treatment tanks (p < 0.001). O3 was measured only in O3 and O3-NB treatments. O3 values of O3-NB treatment were significantly lower than O3 treatment tanks (p < 0.001) in Experiment 1 (Table 2).
Discussion
Our findings suggested that treatments with nanobubbles could result in mild to moderate gill pathology. In our first experiment, the majority of shrimp with gill changes were classified with mild changes, and shrimp were active and had normal growth. Our findings were consistent with other studies reporting supersaturation with oxygen adversely affecting the gill structure of shrimp (Boyd & Fast, 1992; Weitkamp & Katz, 1980) and fish (Tzaneva et al., 2011). A recent study by Jhunkeaw et al. (2021) also did not find mortality and or cell damage in tilapia (Oreochromis niloticus) 48 hours post exposure to a 10 minutes treatment with O3-NB. However, perhaps more frequent shorter exposures to O3-NB would eventually irritate the gills of fish.
The morphological changes observed in our study were more severe in Experiment 2 than in Experiment 1 likely because shrimp were exposed to nanobubbles for a longer period, and they were exposed to bacteria. Experiment 2 suggests the mild damage to gills associated with exposure to O3-NB do not outweigh the benefits that this treatment has on disinfecting water during an outbreak of Vibriosis.
Although it is possible that poor water quality during our treatments could account for some of the gill pathology as nitrogen compounds can result in epithelial oedema, haemolymph infiltration, multifocal melanization and necrosis in shrimp (Fregoso et al., 2017), the values for NH3, NO2, and NO3 in our tanks were within normal range for shrimp.
The result of both experiments suggested that repeated O3 treatments (macrobubble) reduced growth rate of shrimp during our 21 days experiment. However, using O3 in nanobubble form did not affect the growth rate of shrimp. This observation paralleled the gill pathology and the mortality results. In effect, when O3 is delivered using nanobubble it appears to be less toxic and irritating to shrimp than when it was delivered with an aerator (macrobubble). Several water quality parameters differences were observed during the treatment with O3-NB and O2-NB compared to other treatments and these may have contributed to the weight gain differences. For example, the use of nanobubble in tanks increases water temperature by up to 2°C which could have accounted for the weight gain result in our study, however, the changes in weight were not large and were not significant except for the reduction in growth in the O3 macrobubble group compared to the other treatments. The use of O3 treatment without nanobubble appears to have a negative impact on growth of shrimp, so care should be taken when applying this gas using an aerator on commercial farms.
In the absence of bacteria (Experiment 1) the O3 macrobubble treatment had the highest mortality. This finding was supported by Gonçalves & Gagnon (2011) who found that higher ozone concentrations are a risk to farmed species causing gross tissue damage and mortality. However, when O3 was applied in nanobubble form, we reduced the mortality. This implies that O3-NB treatment might be useful for controlling bacteria and increasing the DO without negative impact on shrimp.
When V. parahaemolyticus exponentially increased in the tanks, O3-NB treatment had a disinfection effect, which resulted in significantly lower mortality in shrimp (Experiment 2). Use of O3-NB may cause some gill changes but appears to be beneficial for reducing mortality in the presence of a pathogen. Given the results of Experiment 2 this benefit appears to be due to the reduction in bacteria. It might also be possible to the control bacteria levels without having to treat shrimp every day with ozone nanobubbles, which may further reduce the negative impact on culture species. The result of the study suggested that short frequent O3-NB treatments may be a solution to controlling bacteria, maintain DO content, and limit mortality associated with V. parahaemolyticus in shrimp culture.
The decline in the concentration of V. parahaemolyticus after O3 macrobubble and nanobubble treatments was consistent with our previous published study (Nghia et al., 2021). The application of O3 in nanobubble form seems slightly more efficient at killing bacteria than O3 delivered via macrobubble after 6 hours despite the fact the O3 was delivered for a shorter period than the O3-NB. A study by Ikehata & Li (2018) also found O3 and O3-NB had similar disinfection properties.
The disinfection properties observed in our treatment groups was attributed to high levels of ozone. Ozone levels were higher in the macrobubble group than in nanobubble group, which could explain the negative impact on the shrimp in this study. ORP was higher in the O3 treatments than the O2 treatments. ORP values of about 150–250 mV can promote nitrification, control bacterial growth, but above 350 mV starts killing bacteria (Cefas, 2010; Higgins, 2008). It should be noted that ORP is difficult to measure in water with high organic matter (Suslow, 2004) that reflects lower ORP values in Experiment 2. In water ozone and ORP values are positively correlated (Cefas, 2010; Nghi et al., 2018) so we can validate the accuracy of measurement by comparing their values at the same selected time points.
DO values massively increased during the O2 and O3 treatments, which was similar to what has been reported by Wang et al. (2018). The excessive levels of dissolved oxygen in our O2 treatment groups may have resulted in the gill pathology observed in this group of shrimp. The levels were well above the supersaturation level recommended for shrimp. The O3-NB treatment groups also had elevated DO but did not have severe gill pathology. O3 and O3-NB treatment both increased DO, ORP and decreased NO2 compared with all other treatments; and decreased NO3, NH3, and NH4 compared to the control. The results demonstrate the suitability of applying nanobubble to improve water quality for shrimp farming.
Conclusion
High numbers of shrimp with mild to moderate gill damage were observed in the O3, and O2 (macrobubble) treatments but these lesions were much less common in the O2-NB and O3-NB (nanobubble) treatment groups in both experiments. These results suggest shrimp gills were most affected by the O2 and O3 treatments when these gases were not delivered using nanobubble form. The delivery of these gases using nanobubbles appeared to reduce the negative effect of supersaturation. All treatments except the O3-NB treatment had a higher mortality at the end of the study than the negative control in the Experiment 2. Bacteria counts in water declined quickly in all tanks especially in the two O3 treatments suggested that besides increasing oxygen in the water, the use of O3-NB had the added benefit of reducing the bacterial load in the water and improved shrimp survival. Our study suggests that O3-NB can be applied to shrimp to effectively control V. parahaemolyticus, and hence, reduce AHPND. However, field studies are needed to confirm laboratory results and refine treatments for maximum effectiveness and use of O3-NB in intensive commercial shrimp farms.