Introduction
A tuna cooking juice is one of the byproducts of the canned tuna industry and is estimated to comprise 10% to 14% of liquid byproducts that have long been underutilized (Prasertsan et al., 1988). Indeed, the tuna cooking juice and tuna cooking juice concentrate (TC) are rich in proteins (Ciou et al., 2020). The protein content in tuna cooking juice and TC accounts for 3.75% to 5.65% and 48.91% of the content, respectively, which may serve as potential protein sources. However, the utilizing of TC is limited due to its physical properties, including dark brown coloration, high viscosity, and fishy odor.
Hydrolysis of fish protein helps improving the color and appearance of the juice and removing the fishy odor, and is important for the generation of physiologically and nutritionally important peptides in proteins (Mongkonkamthorn et al., 2021). Protein hydrolysis can be performed through several methods, including chemical and enzymatic hydrolysis. Among these methods, enzymatic proteolysis is the most common because, compared to chemical approaches, the hydrolysis conditions are milder, and the enzyme can cleave specific peptide bonds, resulting in the production of peptides with various sizes and free amino acids (AAs) (Tapal & Tiku, 2019). Several studies have been conducted to elucidate whether protein hydrolysates/bioactive peptides exhibit promising antioxidant (Phongthai et al., 2018), anti-inflammatory (Cheng et al., 2015), and angiotensin I-converting enzyme inhibitory activities (Mongkonkamthorn et al., 2021). These results suggest the suitability of protein hydrolysates/bioactive peptides as functional food ingredients. Therefore, research has been focused on improving the utilization of protein byproducts by producing protein hydrolysates.
Optimization of hydrolysis conditions is critical for the production of desirable protein hydrolysates because the physical and functional properties, especially antioxidant activities, of the hydrolysates are affected by several factors, including the hydrolysis conditions (enzyme concentration, hydrolysis time, pH, and temperature), enzyme types, and substrates (Auwal et al., 2017; Zhuang et al., 2009). When several factors are involved in the optimization process, each factor must be individually examined, which is time-consuming, results in low precision and may lead to a false optimum. Therefore, the design of experiments method is a widely accepted, fast alternative approach due to its wide range of experimental designs, i.e., full factorial design, fractional factorial design, the Taguchi method, and the response surface method (RSM) (Wahid & Nadir, 2013).
RSM is generally used for the optimization of experimental parameters by employing mathematical and statistical techniques for reducing treatment numbers and detecting the effects of experimental parameters (Kumari & Gupta, 2019). RSM has been applied for optimizing the production conditions of protein hydrolysate from several sources, e.g., protein hydrolysate from jellyfish (Rhopilema esculentum) (Zhuang et al., 2009) and skipjack tuna (Katsuwonus pelamis) dark muscle (Mongkonkamthorn et al., 2021). In both cases, hydrolysates were successfully produced with high 2,2-diphenyl-1-picrylhydrazyl (DPPH) radical scavenging activities. These studies suggested that RSM was a potential tool for the optimization of the production conditions of protein hydrolysates. To the best of our knowledge, optimizing the production of TC hydrolysates (TCH) by RSM is limited.
Based on the abovementioned description, the aim of this study was to optimize the hydrolysis conditions to produce TCH with high antioxidant properties by RSM. The enzyme (Alcalase) concentration and hydrolysis time were varied to maximize the DPPH radical scavenging activity of TCH. The effect of different drying methods on the radical scavenging activity by DPPH assay was evaluated. In addition, TCH was further fractionated based on molecular weight, and several antioxidant activities and AA profiles were determined.
Materials and Methods
TC was obtained from a canned tuna processing plant in Samut Sakhon province, Thailand. It was packed in polyethylene bags and transported in an icebox at approximately 4°C to the laboratory (Kasetsart University, Bangkok) within 2 h. After arriving, TC was mixed thoroughly, and a portion was taken for proximate composition (protein, moisture, lipid, and ash) analyses (AOAC, 2000; analytical method No. 920.153, 950.46, 960.39, and 928.08, respectively), carbohydrate content analyses (the content was calculated by subtracting the sum of the protein, moisture, lipid, and ash from 100), and physical property (pH) analyses using an MM-60R pH meter (DKK-TOA, Tokyo, Japan). The remaining portions of TC were stored in airtight bottles at –18°C for subsequent use.
For subsequent color analysis and DPPH radical scavenging activity assay, TC was thawed at 4°C for 24 h, heated at 60°C for 10 min, and maintained at 4°C overnight to promote the stratification of lipids (wax formation above the TC) and insoluble materials (precipitation at the bottom layer of TC) (Kasiwut et al., 2015). The lipids and insoluble materials were separated by cloth filtration. A few portions of the filtrated TC were taken and frozen at –18°C for 48 h prior to freeze drying using a Scanvac Coolsafe Touch 95–15 freeze dryer (Labogene, Lillerød, Denmark) for 74 ± 2 h. The condenser temperature and pressure of the freeze dryer were set at –50°C and 0.002 mbar, respectively. Then, the resulting lyophilized TC was ground and subjected to color analysis using an Ultrascan XE 205 HunterLab colorimeter (Hunter Associates Laboratory, Reston, VA, USA) and DPPH radical scavenging activity analysis as described in the DPPH radical scavenging activity section. The remaining portions of the filtrated TC were used to prepare TCH as described in the following section.
TCH was prepared from TC by Alcalase 2.4 L FG (E.C. 3.4.21.62; Novozymes, Bagsvaerd, Denmark) hydrolysis according to our preliminary trial, the manufacturer’s protocol of proteases for biocatalysis (Novozymes, 2016), and the method described by Dong et al. (2008) with slight modifications. Briefly, TC was diluted with water (1:2 [w/v]) based on our preliminary trial (this sample-to-water ratio resulted in the highest value of DPPH radical scavenging activity [data not shown]). The sample was adjusted to pH 8 by dropwise additions of 0.1 M NaOH under continuous stirring and was preheated at 60°C by incubation in a water bath (Memmert, Bavaria, Germany) for 20 min. Then, enzymatic hydrolysis was performed using Alcalase, following the conditions described in Table 1. After hydrolysis, Alcalase was inactivated by heating the reaction mixture in boiling water at 100°C for 15 min. Then, TCH (supernatant) was obtained after centrifugation at 10,000×g for 15 min at 4°C using a Suprema 21 refrigerated centrifuge (Tomy Seiko, Tokyo, Japan) and was subjected to freeze drying as described in the Raw Material Section.
RSM was used to optimize the production conditions to maximize the DPPH radical scavenging activity of TCH. Central composite design (CCD) was employed by two factors, the Alcalase concentration (X1) and hydrolysis time (X2). Each factor was varied by five levels (coded factor levels at –1.414, –1, 0, 1, and 1.414; uncoded factor levels of X1 at 0.6%, 1%, 2%, 3%, and 3.4% and of X2 at 10.30, 60, 180, 300, and 350 min), and various hydrolysis conditions were predicted, as shown in Table 1. In total, there were 9 conditions and 13 runs (5 runs for the central points). All TCHs were produced and their DPPH radical scavenging activity was analyzed. The relationship between the dependent and independent variables is illustrated in Equation (1). A three-dimensional response surface graph and a contour graph were also plotted to demonstrate the relationship between the levels of independent variables and the outcome or response. Minitab ver. 17 (Minitab, State College, PA, USA) was used for the experimental design, data analysis, and model building in CCD and to generate three-dimensional response surface and contour plots.
where Y is the DPPH radical scavenging activity, X1 is the Alcalase concentration, X2 is the hydrolysis time, β0 is the constant coefficient, β1 and β2 are linear coefficients, β3 is the interaction coefficient, and β4 and β5 are quadratic coefficients.
The TCH that was produced under optimal conditions (Fig. 1A) was spread in an aluminum tray and dried by the following methods: 1) freeze drying, 2) hot air drying, and 3) vacuum drying. Freeze dried TCH (FD) was obtained as described in the Raw Materials Section, and a few portions of the FD were subjected to proximate composition (AOAC, 2000) and physical property analyses. Hot air dried TCH (OD) was obtained using a UN110 hot air oven (Memmert) at 70°C for 80 h. A VO101 vacuum oven (Memmert) set at 70°C and 72 mbar for 76 h was used to obtain vacuum dried TCH (VD). The resultant TCH powders from each of the drying methods (moisture content < 10%) were stored at –18°C for the latter AA analysis, DPPH radical scavenging activity analysis, and ultrafiltration.
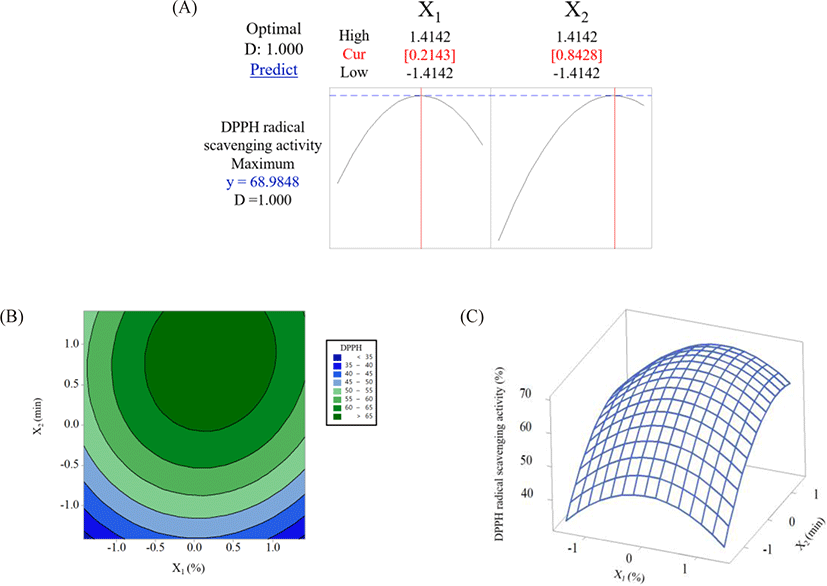
The VD samples were dissolved in deionized sterile water to obtain a final concentration of 100 mg/mL and were subjected to ultrafiltration using two molecular weight cutoff membranes (MWCO; 5 and 10 kDa; Corning, New York, NY, USA). Initially, 14 mL of VD solution (100 mg/mL) was placed in a 50 mL centrifuge tube containing 10 kDa MWCO. The protein was separated using a UNIVERSAL 32R refrigerated centrifuge (Hettich-Zentrifugen, Tuttlingen, Germany) that was set at 6,000×g for 30 min at 4°C. The resulting solutions, above (> 10 kDa) and below (< 10 kDa) the membrane, were collected. The solution below the membrane was subjected to another ultrafiltration using 5 kDa MWCO with the same centrifugation conditions. The following VD fractions were obtained: 1) VD-1 (MWCO < 5 kDa; the fraction collected below the 5 kDa membrane), 2) VD-2 (MWCO = 5 to 10 kDa; the fraction collected above the 5 kDa membrane), and 3) VD-3 (MWCO > 10 kDa; the fraction collected above the 10 kDa membrane) and were vacuum-dried (Memmert) for subsequent AA analysis and antioxidant activity measurements.
The AA content was determined by high-performance liquid chromatography (HPLC; 1200 Infinity Series, Agilent Technologies, Santa Clara, CA, USA) according to the manufacturer’s protocol of automated AA analysis using an Agilent Poroshell HPH-C18 column for AA analysis (Agilent Technologies) as described by Long (2021). Briefly, a 0.5-g portion of TCH or TCH fractions (FD, OD, and VD) was hydrolyzed with 5 mL of 6 M HCl in a B-300 oil bath (Buchi, Flawil, Switzerland). After 24 h of incubation at 110°C, the hydrolyzed samples were diluted with 50 mL of HPLC-grade water (Honeywell Burdick and Jackson, Muskegon, MI, USA) to obtain a final concentration of 10 mg/mL and were filtered through a 0.22 μm nylon membrane filter (Filtrex Technologies, Encinitas, CA, USA). Then, a 10-μL portion of the filtrates was subjected to HPLC analysis. Analytes were separated in a Poroshell-120 column (HPH-C18, 4.6 × 100 mm, 2.7 μm) at 40°C. Mobile phase A consisted of 10 mM disodium hydrogen phosphate (Na2HPO4), 10 mM sodium tetraborate (Na2B4O7 · H2O), and 0.5 mM sodium azide (NaN3) (pH 8.2). Mobile phase B consisted of acetonitrile, methanol, and water (45:45:10 [v/v/v]). The flow rate was set at 1 mL/min. The gradient condition was set at 2% B from 0 to 0.35 min, 57% B at 13.4 min, 100% B from 13.5 to 15.7 min, and 2% B from 15.8 to 18.0 min. Each eluent was detected by a fluorescence detector with excitation and emission wavelengths of 230 nm and 450 nm, respectively. The AA contents of the TCH and TCH fractions were quantified against a standard curve obtained using an AA standard (Agilent Technologies).
The DPPH radical scavenging activity was analyzed according to the methods described by Yen & Hsieh (1995) with slight modifications. Briefly, TC, TCH, or TCH fractions (VD-1, VD-2, and VD-3) were individually dissolved in distilled water (10 mg/mL), and 1 mL of the sample solution was mixed with 1 mL of 85% ethanol containing 200 µM DPPH. The reaction mixture was incubated at room temperature for 30 min in the dark, and the absorbance at 517 nm was determined using an Evolution 300 UV–Vis spectrophotometer (Thermo Scientific, Waltham, MA, USA). Trolox (0 to 1.8 µM) was used as a reference standard. The DPPH radical scavenging activity was calculated as illustrated in Equation (2). Data were expressed as a percentage, µmol Trolox/mg protein, or half maximal inhibitory concentration (IC50).
where Acontrol is the absorbance of the reaction mixture without a sample, and Asample is the absorbance of the reaction mixture with a sample or Trolox.
The 2,2’-azino-bis(3-ethylbenzthiazoline)-6-sulfonic acid (ABTS) radical scavenging activity was determined following the methods described by Alemán et al. (2011) with slight modifications. Briefly, the ABTS radical cation (ABTS•+) was generated in the reaction of 14.8 mM ABTS and 5.2 mM potassium persulfate. After 12 to 16 h of incubation at room temperature in the dark, the absorbance at 734 nm of the ABTS solution was adjusted to 0.700 ± 0.02 by adding 200 mM phosphate buffer, pH 8.2 (1:1 [v/v]). One milliliter of this solution was mixed with 0.1 mL of 200 mM phosphate buffer (pH 8.2) containing an individual TCH fraction (10 mg/mL) and 0.9 mL of deionized water. The mixture was incubated at room temperature for 10 min in the dark. Then, the absorbance at 734 nm was determined by an Evolution 300 UV–Vis spectrophotometer (Thermo Scientific) against Trolox (0 to 200 µM) as a reference standard. The ABTS radical scavenging activity of TCH fractions was reported as µmol Trolox/mg protein.
The ferric reducing antioxidant power was determined following the methods described by Oyaizu (1986) with slight modifications. Briefly, a 1-mL portion of aqueous TCH fractions (10 mg/mL) was mixed with 1 mL of phosphate buffer (200 mM, pH 6.6) and 1 mL of 1% (w/v) potassium ferric cyanide. After incubation at 50°C for 20 min, 1 mL of 10% (w/v) trichloroacetic acid was added to the mixture. A 2.5 mL aliquot of the mixture was taken and mixed with 2.5 mL of deionized water and 0.5 mL of 0.1% (w/v) ferric chloride. Then, the ferric reducing antioxidant power was determined at 700 nm by an Evolution 300 UV–Vis spectrophotometer (Thermo Scientific) against Trolox (0 to 600 µM) as a reference standard. Data were expressed as µmol Trolox/mg protein.
The ferrous ion chelating activity of the TCH fractions was determined according to the method described by Decker & Welch (1990) with slight modifications. Briefly, dried TCH fractions were individually dissolved in distilled water (10 mg/mL). One milliliter of the sample solution was transferred to another tube and mixed with 3.7 mL of distilled water, 0.1 mL of 0.2 mM ferrous chloride, and 0.2 mL of 5 mM ferrozine. After incubation at room temperature for 10 min, the ferrous ion chelating activity was determined by an Evolution 300 UV–Vis spectrophotometer (Thermo Scientific) at 562 nm. EDTA (0 to 0.1 mM) was used as a reference standard, and the ferrous ion chelating activity was calculated as illustrated in Equation (3). Data were expressed as µmol EDTA/mg protein.
where Acontrol is the absorbance of the reaction mixture without a sample, and Asample is the absorbance of the reaction mixture with a sample or EDTA.
The statistical analysis of the regression coefficients was performed by analysis of variance (ANOVA) using Minitab ver. 17. The fitted value predicted by the response regression equation was compared with the experimental values to validate the model. The statistical analysis of the antioxidant activities of TCH fractions was performed by one-way ANOVA using Duncan’s multiple range test in SPSS ver. 26 (SPSS, IBM, Armonk, NY, USA). A p-value of less than 0.05 was considered significantly different. All data for each of the experiments are reported as the mean ± SD (n = 3).
Results and Discussion
The proximate compositions revealed that protein was a major component found in TC; the protein, moisture, lipid, ash, and carbohydrate contents in TC accounted for 48.91%, 27.98%, 0.39%, 21.73%, and 0.99% of the contents, respectively. Our results are in accordance with other concentrated cooking waters derived from different tuna species, which consisted of 40% to 60% protein as a major component (Ciou et al., 2020; Martínez-Montaño et al., 2021). In addition, the hydrolysis of TC by Alcalase resulted in a significant increase (p < 0.05) in the protein and carbohydrate contents to 58.72% and 18.79%, respectively, but a reduction in the moisture, lipid, and ash contents to 6.05%, 0.04%, and 16.40%, respectively. The increase in protein content could be explained by the decrease in the protein molecular weight and increase in the ionizability and hydrophobicity of TCH after enzymatic hydrolysis, resulting in the higher solubility of protein (Wouters et al., 2016). Similar protein increases have been reported in red meat hydrolysates from mackerel tuna (Euthynnus affinis), which increased the protein content to 89.9% when compared to that of nonhydrolyzed tuna red meat (28.3%) (Parvathy et al., 2018). Overall, our findings were in accordance with the report on protein hydrolysates from bighead carp (Hypophthalmichthys nobilis), by which hydrolysis increased the protein content but decreased the lipid, moisture, and ash contents (Alahmad et al., 2022). These results suggest that byproducts of tuna processing, such as TC, may serve as a good source of protein in the production of value-added fish protein hydrolysate.
The physical properties of TC and TCH revealed that they had slightly acidic pH values of 5.86 and 5.48, respectively. TC had a brown coloration (L* = 14.04, a* = 35.34, and b* = 24.17). However, after enzymatic hydrolysis, the color appearance of TCH was improved as a lighter brown coloration was observed (L* = 32.58, a* = 1.48, and b* = 1.75) (data not shown). Of note, L* was increased, while both a* and b* were decreased in TCH compared to TC, which was in accordance with the findings of Banjongsinsiri et al. (2017). The lighter coloration after hydrolysis was probably due to the degradation of color pigments to colorless compounds. In addition, the differences in peptide size and AA composition between TC and TCH are probably responsible for the color change (Banjongsinsiri et al., 2017).
RSM revealed the effects of the Alcalase concentration and hydrolysis time on the DPPH radical scavenging activity of TCH. Following the experimental conditions described in Table 1, the results showed that the DPPH radical scavenging activity of TCH ranged from 44.54% to 68.26% (IC50 = 1.71 to 2.09 mg/mL). The best production conditions for TCH with DPPH radical scavenging activity under CCD were at 2% (w/v) Alcalase and a hydrolysis time of above 180 min, resulting in a DPPH radical scavenging activity of approximately ≥ 63% (Table 1). The model efficiency of the DPPH radical scavenging activity of TCH was validated (data not shown). Data were linearly distributed, indicating that there were no aberrates in the residual of the results. Therefore, it was concluded that the data had a normal distribution, which was in accordance with the findings of Bahari et al. (2020), in which the data plot of the DPPH radical scavenging activity obtained for sea cucumber (Actinopyga lecanora) hydrolysate was normally distributed. Furthermore, the residuals of the results were evenly distributed between positive and negative values, indicating that the data were independent with stable variance (data not shown).
The ANOVA of the TCH was investigated and is shown in Table 2. The correlation coefficient (R2) of 0.9056 indicated that the statistical model was adequate and could represent the relationships between the Alcalase concentration (X1) and hydrolysis time (X2) on the DPPH radical scavenging activity (Y) of TCH. According to Narkprasom et al. (2015), R2 should be close to 1.0, but a value greater than 0.8 is acceptable. The coefficient of variation (CV) was used to describe the dispersion of the data and to represent the precision and reliability of the results. A lower CV value indicates low variation in the mean value of the dataset. The CV value obtained in this study was 15.06% (Table 2), which is quite low, suggesting that the experimental values have good precision and reliability (Šumić et al., 2016). The suitability of the regression equation was assessed based on the lack of fit value, wherein no significant differences were observed for the lack of fit value, suggesting that the regression equation was suitable. The results obtained in this study showed a significant difference in DPPH radical scavenging activity (p < 0.05) without a significant difference (p > 0.05) in the lack of fit values (Table 2). Our results agreed well with the finding of Halim & Sarbon (2017), who investigated the model to produce eel (Monopterus sp.) protein hydrolysate; significant differences (p < 0.05) were observed for the DPPH radical scavenging activity but not for the lack of fitness (p > 0.05). These results indicated that the regression equation and the fitness of the data are suitable.
Both independent variables (Alcalase concentration and hydrolysis time) had positive linear and interactive relationships with the DPPH radical scavenging activity. However, quadratic effects revealed negative correlations with DPPH radical scavenging activity, resulting in a curvilinear regression Equation (4) as follows:
where Y is the DPPH radical scavenging activity, X1 is the Alcalase concentration, and X2 is the hydrolysis time.
The contour plot and three-dimensional plot and contour plot for the DPPH radical scavenging activity are shown in Fig. 1B and 1C , respectively. These plots show the effects of independent variables (Alcalase concentration and hydrolysis time) on the dependent variable (DPPH radical scavenging activity of TCH). The obtained results showed that an increase in the Alcalase concentration and hydrolysis time increased the DPPH radical scavenging activity of TCH. However, an increase in both variables beyond the optimal point resulted in the reduction of DPPH radical scavenging activity (Fig. 1A). When the enzyme concentration and hydrolysis time increased, the DPPH radical scavenging activity increased, which was probably due to the occurrence of more hydrolysis reactions and the formation of short chained peptides that contained free radical scavenging activity, respectively (Mahdavi-Yekta et al., 2019; Wang et al., 2017). On the other hand, the reduction in the DPPH radical scavenging activity of TCH beyond the optimal point could be explained as follows: 1) the progression of hydrolysis caused the degradation of some antioxidant peptides that had formed at the early stage of hydrolysis and 2) the ratio of enzyme-to-substrate was insufficient for the latter hydrolysis reaction, leading to a reduction in the antioxidant activity of TCH under excess enzyme concentration and hydrolysis time (Mahdavi-Yekta et al., 2019). Similar findings were observed in the study by Wang et al. (2017) and Mongkonkamthorn et al. (2021), in which the DPPH radical scavenging activity of chub mackerel (Pneumatophorus japonicus) and skipjack tuna (K. pelamis) dark muscle protein hydrolysate increased when the enzyme concentration and hydrolysis time increased but gradually decreased when the enzyme concentration and hydrolysis time were excessive.
The raw TC exhibited a DPPH radical scavenging activity of 35.21% (data not shown). After the CCD, the production of TCH under optimal conditions was predicted at 2.2% Alcalase and a hydrolysis time of 281 min (factor levels at 0.2143 and 0.8428, respectively) (Fig. 1A). The validation of the TCH produced under this condition revealed a 66.49% (0.98 µmol Trolox/mg protein) DPPH radical scavenging activity (data not shown). The DPPH radical scavenging activity increased up to 88.84%. In addition, the difference between the predicted (68.98%) and experimental (66.49%) values had a 3.74% error, which was within the acceptable range (5% error). Therefore, the experimental model was accurate within the 95% prediction interval (Choudhary & Pramanik, 2021). The IC50 of TCH produced under the optimal conditions was 1.52 mg/mL (1.37 µmol Trolox/mg protein), which is comparable to that of skipjack tuna (K. pelamis) dark meat hydrolysate (1.1 mg/mL) when optimized by RSM (Mongkonkamthorn et al., 2021).
Drying techniques have significant effects on the rheological, physical, and structural traits of protein hydrolysates. The difference in rheological and physical attribute changes in the AA profiles and functionality provides strong insights into protein hydrolysates, such as their antioxidant activity (Dabbour et al., 2022; Uribe et al., 2020). In this part of the study, the effects of drying methods (freeze drying, hot air drying, and vacuum drying) on the AA profiles, which may consequently affect the DPPH radical scavenging activity of TCH, were investigated.
Per 100 g of protein in all TCHs prepared by different drying methods, cysteine was a major AA component (19.95 to 20.67 g), followed by glycine (14.69 to 15.61 g), glutamic acid (10.25 to 10.80 g), arginine (7.75 to 7.85 g), and alanine (7.32 to 7.76 g). Conversely, tyrosine (1.00 to 1.23 g), isoleucine (1.40 to 1.69 g), and methionine (1.63 to 1.88 g) were observed in the lowest amounts (Table 3). A significant difference (p < 0.05) in AA content with different drying methods was found in glutamic acid, serine, and histidine (Table 3). The highest amounts of glutamic acid and serine content were found in VD, whereas the highest histidine content was found in OD. Some AA contents were less affected by the different drying methods; threonine levels in VD were significantly higher (p < 0.05) than those in FD and OD. Cysteine was significantly higher (p < 0.05) in VD and FD than in OD. Glycine and alanine were significantly higher (p < 0.05) in VD and OD than in FD. The aspartic acid, arginine, tyrosine, phenylalanine, leucine, lysine, and proline contents were independent (p > 0.05) of the drying method. By grouping these AAs, a significantly higher (p < 0.05) abundance of negatively charged AAs (NCAAs) was found in VD than in FD. The aromatic AAs (AAAs) in VD and FD were significantly higher (p < 0.05) than those in OD. Essential AAs (EAAs) were significantly higher (p < 0.05) in FD and OD than in VD. Positively charged AAs (PCAAs) were significantly higher (p < 0.05) in OD than in FD and VD. Hydrophobic AAs (HAAs) were not affected (p > 0.05) by the different drying methods.
Different thermal treatments may improve, maintain, or reduce the AA content in the hydrolysate depending on the initial AA content or the drying conditions (Uribe et al., 2020). Because the initial AA content in TCH before being subjected to different drying methods was the same, it should not be considered a factor that affects the AA content after drying in this study. However, different drying conditions, i.e., temperature (–50°C for freeze drying or 70°C for hot air drying and vacuum drying) and oxygen concentrations (atmospheric oxygen for hot air drying or vacuum for freeze drying and vacuum drying), played an important role in the AA profiles of hydrolysate. High temperature can increase the AA content due to the possible progression of proteolysis during drying (Uribe et al., 2020). Vacuum conditions, which involve an oxygen-free environment, can prevent compounds from undergoing oxidative degradation (How & Siow, 2020; Jakkranuhwat & Kunchansombat, 2021). Based on the obtained results, we proposed that changes in the AA profiles of TCH under different drying conditions may be due to these reasons.
DPPH is one of the most stable and general organic nitrogen radicals that is commercially available, and it can accept an electron or hydrogen ion and form a stable molecule (Tang et al., 2013). In this study, DPPH activity was altered by the different drying methods. The DPPH radical scavenging activity of VD was significantly higher (p < 0.05) than that of OD and FD (1.20, 1.07, and 0.98 µmol Trolox/mg protein, respectively), although there was no significant difference (p > 0.05) in HAA, which plays an important role in the radical scavenging activity of peptides among treatments (Fig. 2 and Table 3). This was probably due to the protection of the antioxidants present in VD under vacuum conditions (oxygen-free environments) (How & Siow, 2020; Jakkranuhwat & Kunchansombat, 2021). In addition, higher temperatures and longer drying times under a vacuum may lead to the alteration of the structural conformation of peptides. Consequently, the formation of new compounds with antioxidant activities, such as melanoidins derived from the Maillard reaction, might occur (Uribe et al., 2020). These factors caused VD to exhibit a significantly higher (p < 0.05) DPPH radical scavenging activity than that of FD and OD, which lacked the heat treatment and oxygen-free conditions, respectively. The results of this study were consistent with the finding of Uribe et al. (2020), wherein vacuum drying, which uses heat treatment and vacuum conditions, remarkably increases the DPPH radical scavenging activity of brown algae (Durvillaea antarctica) compared to that of various drying methods. Therefore, we recommend vacuum drying as a suitable drying method to produce protein hydrolysates that exhibit high DPPH radical scavenging activity.
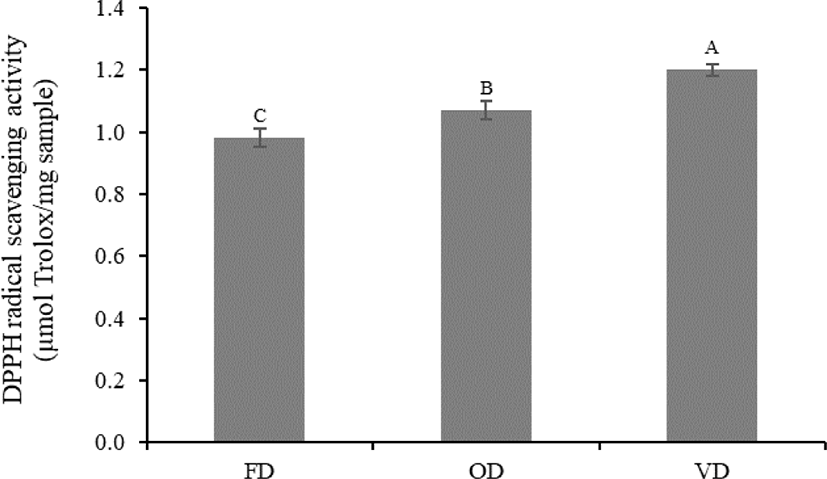
VD was subjected to ultrafiltration using 3 MWCO. The following VD fraction were obtained: VD-1 (MWCO < 5 kDa), VD-2 (MWCO = 5 to 10 kDa), and VD-3 (MWCO > 10 kDa). The predominant AA contents in all VD fractions per 100 g protein were 13.24 to 15.17, 12.19 to 13.76, 10.26 to 11.10, and 8.95 to 12.03 g for cysteine, glycine, glutamic acid, and arginine, respectively. A high yield of these amino products was obtained, which can be attributed to enzymatic hydrolysis (specifically by Alcalase). The results obtained in this study were in accordance with the previous finding by Henriques et al. (2021), in which hydrolysis by Alcalase resulted in high levels of glutamic acid, glycine, and arginine from protein byproducts hydrolysates in gurnard fish (Trigla spp.). Additionally, a high yield of glutamic acid was also associated with a high content of glutamic acid in tuna (Thunnus albacares and Thunnus obesus) meat (Peng et al., 2013). However, the high contents of cysteine in our study differed from other findings (Henriques et al., 2021). This might be due to the high content of cysteine that originally existed in TC (approximately 21 g/100 g protein), and this was different from fish protein byproducts hydrolysate, which had low contents of cysteine (approximately 0.4 g/100 g protein).
Aside from cysteine and glycine, enzymatic hydrolysis by Alcalase may also produce other HAAs, such as alanine, leucine, isoleucine, and proline (Tacias-Pascacio et al., 2020). The presence of HAA may improve the solubility of peptides in hydrophobic environments. This helps simplify interactions between peptides and helps donate protons to radical species. Thus, hydrophobic interactions among HAA residues may enhance physicochemical and functional properties, such as the antioxidant activity of peptides (Karimi et al., 2020).
Significant changes in AA contents after fractionation were observed in cysteine, glycine, arginine, histidine, and proline (Table 4). Cysteine and glycine were present at significantly higher amounts (p < 0.05) in the larger fraction (VD-3) than in the smaller fractions (VD-1 and VD-2). Arginine and proline were significantly higher (p < 0.05) in VD-1 than in VD-2 and VD-3. Histidine was significantly higher (p < 0.05) in VD-2 than in VD-1 and VD-3. The remaining AA contents were not affected (p > 0.05) by fractionation. By grouping the AAs, the HAA, AAA and NCAA contents of VD-3 were found to be the highest among fractions, accounting for 52.11, 3.81, and 16.57 g/100 g protein, respectively. The highest content of PCAA was found in VD-2 at 25.24 g/100 g protein. EAA, a category of AAs that cannot be synthesized in humans alone, was provided at high amounts by each of the VD fractions (Table 4). The EAA content (except for undetected tryptophan, which cannot be hydrolyzed by acid) accounted for 27.13, 29.29, and 26.53 g/100 g sample in VD-1, VD-2, and VD-3, respectively. These amounts were slightly different but were comparable to the standard set by the Food and Agriculture Organization/World Health Organization for the dietary intake of EAA for adults (27.70 g/100 g of protein) (FAO/WHO, 2007). However, of note, unlike the FAO/WHO standard, the amount of EAA in the VD fractions reported in this study lacked tryptophan.
Different uppercase letters indicate significant differences among the mean value of each treatment (p < 0.05).
AA, amino acid; VD, vacuum dried tuna cooking juice concentrate hydrolysate; VD-1, VD with a molecular weight cutoff < 5 kDa; VD-2, VD with a molecular weight cutoff range of 5 to 10 kDa; VD-3, VD with a molecular weight cutoff > 10 kDa; NS, not significant; TAA, total AAs; EAA, essential AAs; HAA, hydrophobic AAs; AAA, aromatic AAs; NCAA, negatively charged AAs (acidic AAs), PCAA, positively charged AAs (basic AAs).
The antioxidant properties of the TCH fractions were evaluated by DPPH radical scavenging activity, ABTS radical scavenging activity, ferric reducing antioxidant power, and ferrous ion chelating activity assays. VD-1 exhibited the highest DPPH radical scavenging activity among the fractions (0.89 µmol Trolox/mg protein), followed by VD-2 and VD-3, respectively (p < 0.05). In contrast, significant ABTS radical scavenging activity and ferric reducing antioxidant power were observed in VD-3 (29.74 and 1,511.1 µmol Trolox/mg protein, respectively), followed by VD-2 and VD-1, respectively (p < 0.05). Significant ferrous ion chelating activity was detected in VD-1 and VD-2 (0.0824 and 0.0829 µmol EDTA/mg protein, respectively) compared with VD-3 (p < 0.05) (Fig. 3). These results suggested that molecular weight plays an important role in the antioxidant activities of peptides (Azemi et al., 2017). From the results, the DPPH radical scavenging activity and ferrous ion chelating activity showed a negative correlation with peptide size, whereas that of ABTS radical scavenging activity and ferric reducing antioxidant power showed a positive correlation with peptide size (Fig. 3). Our results were in agreement with the findings of several studies, wherein similar correlations between peptide size and several antioxidant activities were observed (He et al., 2013; Khositanon et al., 2021; Tang et al., 2010). However, some of our results revealed contradictory correlations between the peptide size and antioxidant activities (Zhao et al., 2011). From these findings, it could be inferred that other factors might be involved.
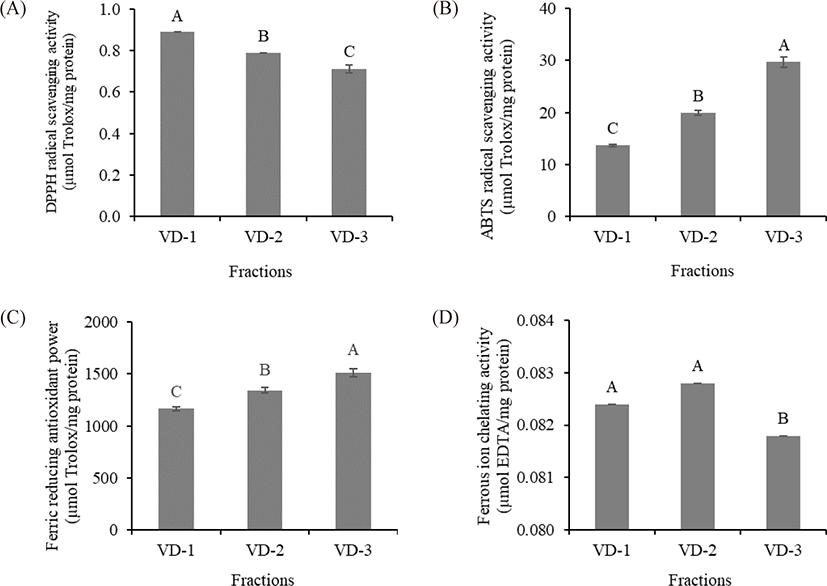
In addition to peptide size, AA profiles may play an important role in the antioxidant activities of VD fractions. Some AA residues in peptides are known to have considerable free radical scavenging activity. The presence of glycine, glutamic acid, and alanine may have promoted peptide interactions with lipids and consequently improved the stability of free radicals (Zamorano-Apodaca et al., 2020). Proline can scavenge free radicals by acting as a hydrogen donor (Zou et al., 2016). Arginine enhances the DPPH and ABTS radical scavenging activities of peptides (Yao et al., 2021). Cysteine and its disulfide molecule (cysteine/cysteine disulfide) affect the antioxidant capacity of peptides by inhibiting the formation of the DPPH complex. Cysteine and its radical can also scavenge ABTS•+, i.e., 1 molecule of cysteine reacts with 1.9 molecules of ABTS•+ (Kim et al., 2020; Zheng et al., 2016). In addition, two phenylalanine and two histidine residues may potentially increase the antioxidant ability of peptides (Zou et al., 2016). Thus, all hydrolysates containing peptides that can react with the radicals and convert them to more stable products can terminate the radical chain reaction. In this study, cysteine, glycine, arginine, histidine, and proline were significantly different (p < 0.05) among fractions. Our results were consistent with the finding of Chaijan et al. (2021), who reported that a high content of these AAs enhanced the DPPH and ABTS radical scavenging activity of peptides. However, significant DPPH and ABTS radical scavenging activities were observed from different fractions. VD-1 exhibited the highest DPPH radical scavenging activity, while VD-3 exhibited the highest ABTS radical scavenging activity. Based on our results, it is likely that not only the AA contents affected the free radical scavenging activity of the VD fraction. Other factors, such as AA sequences, the stereoselectivity of radicals, and the stoichiometry between radicals and antioxidants, may be associated with antioxidant activities (Borawska et al., 2015; Chaijan et al., 2021).
In this study, the sum of AAA (tyrosine and phenylalanine) and PCAA (histidine, arginine, and lysine) content was the highest in VD-1, followed by VD-2 and VD-3, respectively (28.76, 28.54, and 24.33 g/100 g protein, respectively). This resulted in the highest ferric reducing antioxidant power of VD-3 due to the negative correlation between AAA and PCAA on ferric reducing antioxidant power, which was consistent with the finding of Udenigwe & Aluko (2011). Furthermore, ferrous ion chelating activity depends not only on peptide size but also on the presence of specific peptide structures (Phongthai et al., 2018); the AA or carboxyl groups present in the NCAA (aspartic acid and glutamic acid) and PCAA side chain can chelate the transition metal ions by forming strong ionic interactions (Chuesiang & Sanguandeekul, 2015). Specifically, the presence of these AAs in the C-terminal end promotes the ferrous ion chelating activity of peptides (Zou et al., 2016). These data explained why VD-1 and VD-2, which had higher quantities of NCAA and PCAA contents, had higher ferrous ion chelating activities than that of VD-3 (p < 0.05). Similar findings were observed in the study by Chuesiang & Sanguandeekul (2015), in which an increase in the sum of these NCAA and PCAA increased the ferrous ion chelating activity of frame protein hydrolysate from tilapia (Oreochromis nilotica).
Overall, the results suggested that not only peptide size and AA profiles play important roles in the antioxidant activities of TCH fractions, but other factors may also be involved. Therefore, investigations associated with peptide structure would be advantageous for future studies to confirm the sequence and position of AAs in peptides, which may be correlated with various antioxidant activities.
Conclusion
RSM revealed that the optimal hydrolysis conditions for TCH production were 2.2% Alcalase and a hydrolysis time of 281 min; under these conditions, the highest DPPH radical scavenging activity (66.49%) was achieved. Among the drying methods, the vacuum process markedly increased the DPPH radical scavenging activity of TCH. After ultrafiltration, the highest DPPH radical scavenging activity and ferrous ion chelating activity were found in VD-1 (MWCO < 5 kDa). On the other hand, the strongest ABTS radical scavenging activity and ferric reducing antioxidant power were found in VD-3 (MWCO > 10 kDa). Therefore, TC could serve as a potential protein source of raw material in the production of TCH and has promising antioxidant activities for future application as a functional ingredient.