Background
Seahorse is a well-known ingredient in traditional Chinese medicine and is used as an invigorant for the treatment of erectile dysfunction, impotence, wheezing, and nocturnal enuresis. Modern scientific research has proven the pharmaceutical effects of seahorse. Hippocampus kuda has various bioactivities such as anti-tumor, anti-aging, and anti-fatigue as well as Ca2+ channel blocking properties (Kumaravel et al. 2010). A peptide derived from H. kuda has been shown to be effective in chondrocytes and inflammatory arthritis (Kumaravel et al. 2012). In addition, seahorses have a putative free radical scavenging effect in controlling aging process (Kumaravel et al. 2012). However, the natural source of seahorse has dramatically reduced owing to overfishing, unsustainable trade, and habitat destruction (Qian et al. 2012). Therefore, seahorses became the first commercially valuable marine genus to be protected and included in Appendix II of the Convention on International Trade in Endangered Species (CITES) in 2004 (Segade et al. 2015).
Hippocampus abdominalis is one of the largest seahorse species growing up to 35 cm in length (Perera et al. 2016). It was validated for use as a food ingredient by the Ministry of Food and Drug Safety in February 2016. However, the validation was restricted to 50% of the entire composition. For use in food, we should try to prepare a mixture of H. abdominalis by adding other materials. In addition, biological activities of H. abdominalis have rarely been reported so far.
Fatigue is a common distressing condition accompanied by a feeling of extreme physical or mental tiredness that often results in diverse disorders such as anemia, thyroid dysfunction, premature aging, and depression. It could also have adverse effects on work efficiency, physical activities, life quality, and social relationships (Huang et al. 2011). Fatigue is caused by sleep deprivation, inadequate rest, low mood, stress, nutritional imbalance, insufficient exercise, as well as side effects of medications. Chronic fatigue is a persistent unexplainable fatigue lasting for more than 6 months, and it is considered a complex symptom of various neurological, psychiatric, and systemic diseases (Huang et al. 2014). Recently, many researchers have presented the results on the anti-fatigue activity of natural products (Yu et al. 2008; Zhang et al. 2006). Especially, red ginseng has been mainly focused on its anti-fatigue activity with the ability mitigating exercise-related muscle damage, maintaining homeostasis of the body and enhancing vital energy (Kim et al. 2013; Kim et al. 2016). On the other hand, anti-fatigue activity of seahorse has not been scientifically proven although seahorse is a well-known traditional Chinese medicine.
Oxidative stress is caused by an imbalance between reactive oxygen species (ROS) and antioxidant molecules. Excess accumulation of ROS causes oxidative damage by reacting with biomolecules including DNA, membrane lipids, cellular proteins, and diverse pathological states (Kang et al. 2013). Oxidative stress and ROS are the most important causes of exercise-induced disturbances (Fan et al. 2016). In particular, an oxidative imbalance in the skeletal muscle results in increased muscle fatigability. Thus, antioxidants can be used to alleviate fatigue by counteracting the oxidative stress (Nam et al. 2016).
In this study, the effect of H. abdominalis on muscles was investigated to scientifically verify its potential bioactivity. Also, the anti-fatigue activity of a mixture comprising H. abdominalis and red ginseng was investigated to evaluate the synergy effect and to utilize H. abdominalis in the food market. The anti-fatigue activity of H. abdominalis and a mixture was evaluated by measuring the levels of physical fatigue-related biomarkers such as serum glycogen and ATP contents.
Methods
H. abdominalis was kindly donated by Corea Center of Ornamental Reef & Aquariums CCORA (Jeju, Korea) and lyophilized at −70 °C using a freeze dryer. The lyophilized H. abdominalis powder was stored at −80 °C until use. Red ginseng extract containing 30% saponin was purchased from ILHWA Co., LTD. (Gyeonggi, Korea) and lyophilized at −70 °C using a freeze dryer. The lyophilized red ginseng powder was stored at −80 °C until use. Alcalase, a commercial food-grade protease, was purchased from Novozyme Co. (Novozyme Nordisk, Bagsvaerd, Denmark). The other chemicals and reagents used were of analytical grade.
The enzymatic hydrolysis of H. abdominalis was performed using Alcalase under optimal conditions (50 °C and pH 8). The dried H. abdominalis powder was homogenized in distilled water and hydrolyzed using the enzyme at an enzyme/substrate (E/S) ratio of 1:100 for enzymatic reactions. The optimal pH of the homogenates was adjusted before enzymatic hydrolysis. The mixture was incubated for 24 h at the optimal temperature for each homogenate, with stirring, and then boiled for 10 min at 100 °C to inactivate the enzyme. After filtration, all hydrolysates were stored at −70 °C for further experiments.
The H. abdominalis mixtures were prepared by adding different concentrations of red ginseng (RG). The lyophilized H. abdominalis powder and RG powder were mixed as indicated in Table 1, and these seven mixtures were labeled as % of RG.
HH (%) | RG (%) | The mixture (%) |
---|---|---|
80 | 20 | 100 |
70 | 30 | 100 |
60 | 40 | 100 |
50 | 50 | 100 |
40 | 60 | 100 |
30 | 70 | 100 |
20 | 80 | 100 |
The C2C12 myoblasts obtained from American Type Culture Collection (ATCC, Manassas, VA, USA) were cultured in Dulbecco’s Modified Eagle Medium (DMEM) supplemented with 10% heat-inactivated fetal bovine serum (FBS), streptomycin (100 mg/mL), and penicillin (100 u/mL) at 37 °C in a 5% CO2 humidified incubator. To induce differentiation, 80% confluent cultures were switched to DMEM containing 2% horse serum (HS) for 6 days with medium changes every other day.
The cytotoxicity of the samples on C2C12 myoblasts was determined by colorimetric MTT assays. The cells were seeded at 5 × 104 cells per well into 48-well plates. After the induction of differentiation, the cells were treated with various concentrations of the sample and incubated for an additional 24 h at 37 °C. MTT stock solution (100 μL; 2 mg/mL in PBS) was then added to each well. After incubating for 4 h, the plate was centrifuged at 500 g for 10 min, and the supernatant was aspirated. The formazan crystals in each well were dissolved in dimethyl sulfoxide (DMSO). The amount of purple formazan was determined by measuring the absorbance at 540 nm.
The cell proliferation effect of the samples on C2C12 myoblasts was determined by using 5-bromo-2′-deoxyuridine (BrdU) assay (Millipore, Billerica, MA, USA). The cells were seeded at 1 × 104 cells per well into 48-well plates. After the induction of differentiation by switching media, the cells were treated with various concentrations of the sample and incubated for an additional 72 h at 37 °C. Then, the cell proliferation was determined by BrdU reagent following manufacture protocol. In briefly, 10 μL of BrdU reagent was added to each well and the cells were incubated for 2 h. After incubation, the cells were fixed using 100 μL fixing solution. Then, the cells were washed using wash buffer and 50 μL of anti-BrdU monoclonal was added to each well and the cells were incubated for 1 h at RT. The cells were washed using wash buffer and 50 μL of goat anti-mouse IgG was added to each well, and the cells were incubated for 30 min at RT. Also, 50 μL of TMB substrate was added to each well and then, 50 μL of stop solution was added to each well. Finally, the cell proliferation was calculated by comparison with the absorbance at 450 nm of standard solutions of BrdU in the non-treated cells.
The anti-fatigue activity was determined by measuring cell proliferation as well as the glycogen, ATP contents in H2O2-treated C2C12 myoblasts. The cells were seeded into 48-well plates. Then, they were treated with various concentrations of the sample during the differentiation period. After differentiation, fatigue was induced by adding H2O2 to each well at a concentration of 100 μM; then, the cells were incubated for an additional 24 h at 37 °C.
To investigate the effect of the samples on muscle growth, we determined several factors such as glycogen and ATP contents in C2C12 myoblasts. For analysis of the effects of the sample on glycogen accumulation, the glycogen content in the cells was measured via glycogen assay (Abcam, Cambridge, MA, USA). Glucoamylase hydrolyzes glycogen to glucose, which was then specifically oxidized to form an intermediate product that reacts with OxiRed probe to generate color. The color was detected by measuring the absorbance at 450 nm. To determine ATP contents, the cell lysates were deproteinized with 4 M perchloric acid (PCA) and 2 M KOH, and the supernatant was assessed using ATP assay kits (Abcam, Cambridge, MA, USA).
Results and discussion
Cell viability was estimated using the MTT assay, which is a test of metabolic competence predicated upon the assessment of mitochondrial performance. It is a colorimetric assay, which is dependent on the conversion of yellow tetrazolium bromide to its purple formazan derivative by mitochondrial succinate dehydrogenase in the viable cells (Kang et al. 2012). The viabilities of C2C12 myoblasts treated with different concentrations of HH (50, 100, 150, and 200 μg/mL) were expressed to represent 100% viability (the viability of control cells; Fig. 1). In a preliminary experiment, HH concentrations up to 200 μg/mL showed no significant cytotoxicity for 24 h.
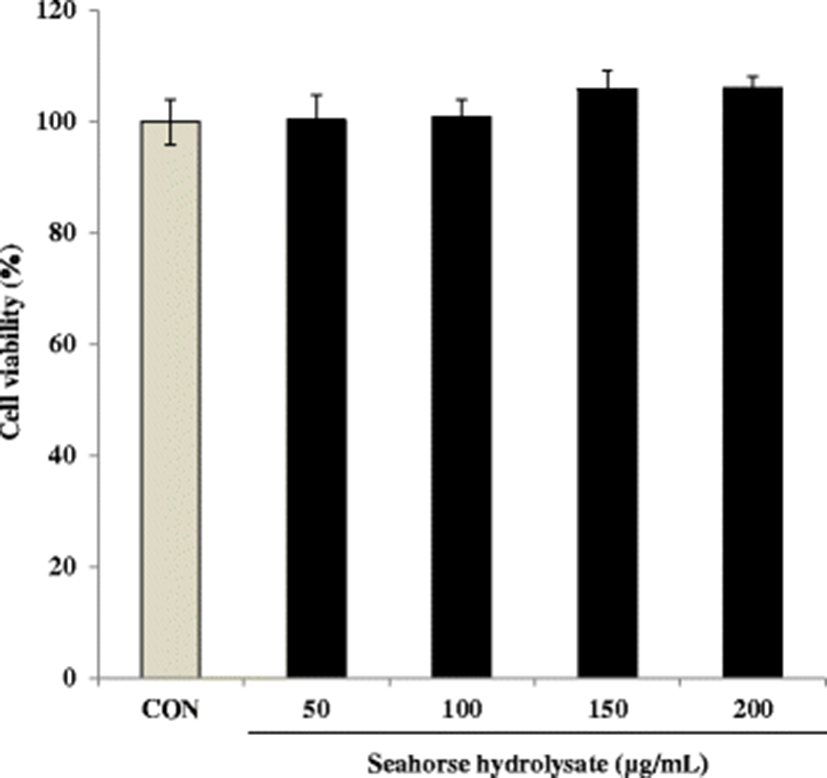
HH significantly enhanced cell proliferation in C2C12 myoblasts compared with the control cells (Fig. 2). In particular, HH treatment induced cell proliferation in a concentration-dependent manner in the range of 100–200 μg/mL. The cell numbers increased approximately 1.8-fold by HH treatment at 200 μg/mL concentration (Fig. 2).
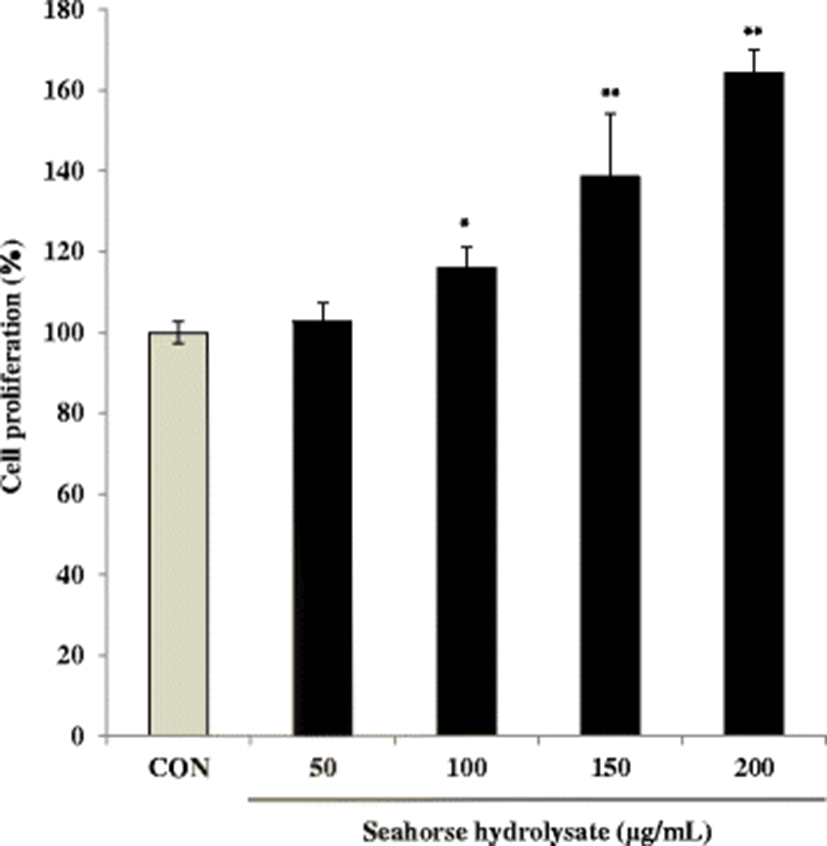
The skeletal muscles are the major site of glycogen storage in the body (Deshmukh et al. 2015). The glycogen content in C2C12 myoblasts was increased by HH treatment at concentrations of 50 and 100 μg/mL (Fig. 3). In C2C12 myoblasts, HH (100 μg/mL) increased glycogen content by 1.5-fold compared with that in the control cells. However, HH treatment at higher concentrations (150 and 200 μg/mL) decreased glycogen content. Thus, it can be suggested that high concentrations of HH suppressed glycogen content.
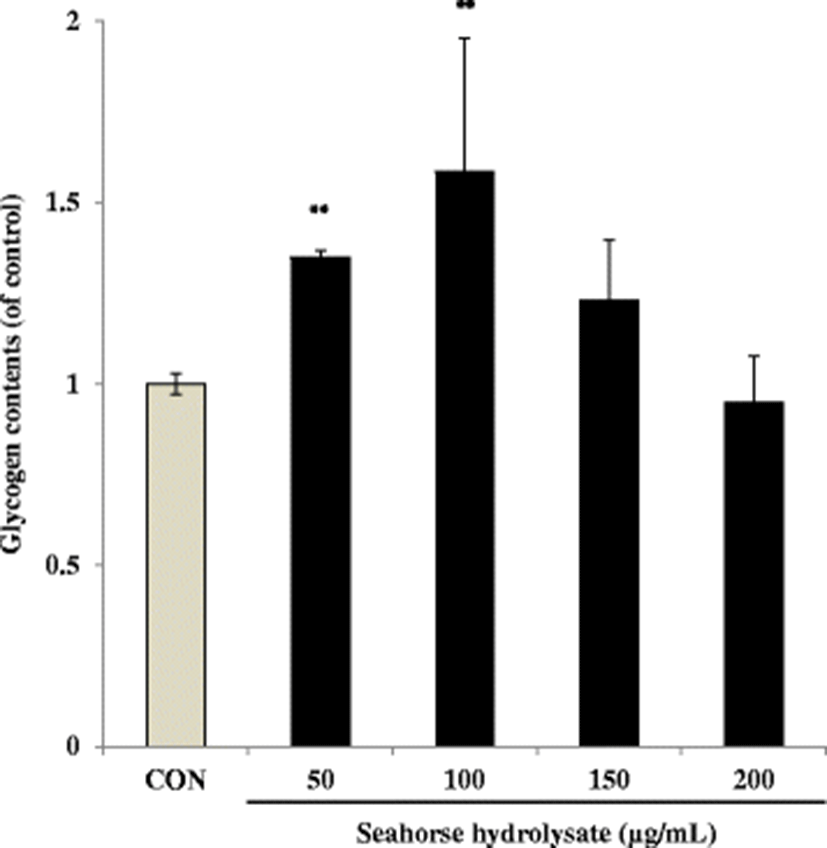
Although H. abdominalis was validated for use in food by the Ministry of Food and Drug Safety in February 2016, the validation was restricted to 20% of the entire composition. To use H. abdominalis as a food ingredient, we should prepare an H. abdominalis mixture by adding other materials.
RG has been frequently used in traditional Asian medicine to treat many disorders, such as debility, aging, stress, diabetes, and insomnia (Tang et al. 2008). Especially, RG has been mainly focused on its anti-fatigue activity with the ability mitigating exercise-related muscle damage, maintaining homeostasis of the body, and enhancing vital energy (Kim et al. 2013; Kim et al. 2016). Thus, H. abdominalis mixtures were prepared by adding different concentrations of RG (20, 30, 40, 50, 60, 70, and 80% of RG) to investigate the synergy effect between H. abdominalis and RG on anti-fatigue activity.
Effect of these mixtures on cell viability was estimated using the MTT assay. The viabilities of C2C12 myoblasts treated with the mixtures at different concentrations (50, 100, 200, 250, and 500 μg/mL) were expressed to represent over 90% viability, which was similar to that of the control cells (Fig. 4). Thus, mixtures up to 500 μg/mL concentration did not show any significant cytotoxicity for 24 h.
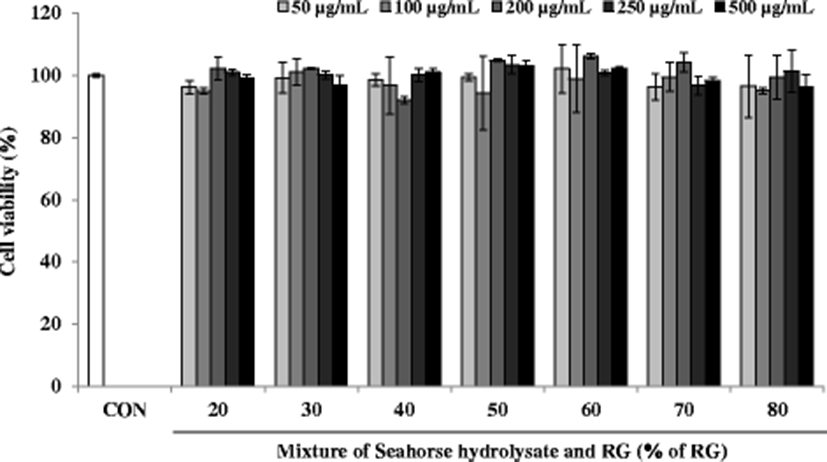
To assess the effect of HH (0% of RG) and RG mixture on muscle growth, cell proliferation was measured as shown in Fig. 5. HH and RG mixtures showed the significant effect on cell proliferation. Especially, at the low concentrations (200 and 250 μg/mL), HH significantly enhanced proliferation of C2C12 myoblasts compared with that of mixtures as well as that of the control cells. However, at the high concentrations (400 and 500 μg/mL), HH and RG mixtures did not show any significant effects on cell proliferation to each other.
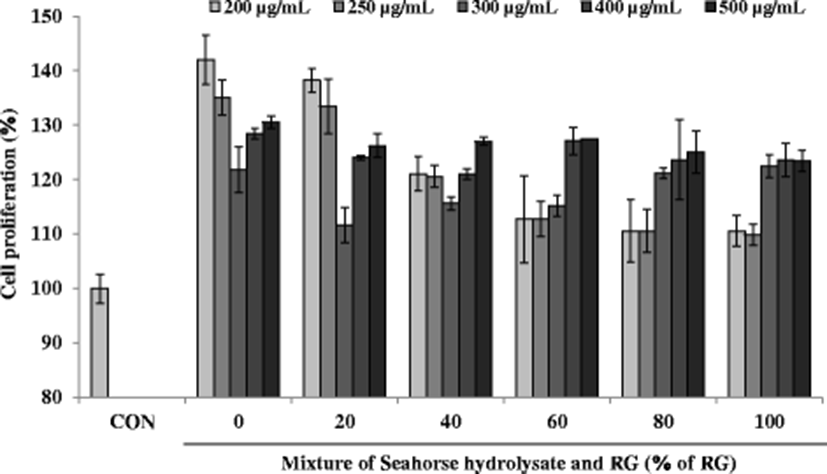
Several biomarkers such as lactate dehydrogenase (LDH), glycogen, aspartate transaminase (AST), and alanine transaminase (ALT) have been used to investigate muscle injury during exhaustive exercise (Huang et al. 2015). Also, fatigue is related to mitochondrial dysfunction and diminished ATP levels (Singh and Singh 2014). The anti-fatigue activity of the mixtures of HH and RG was assessed in H2O2-treated C2C12 myoblasts by measuring cell proliferation as well as the glycogen and ATP contents. Severe and continuous exercise may elevate the formation of ROS, thereby increasing oxidative stress. A sustained elevated oxidative stress can hamper mitochondrial function resulting in low ATP synthesis and increased lactic acid in the muscles, consequently decreasing the physical efficiency. These observations suggest that improving the antioxidant status may enhance the overall physical performance by maintaining the pro-oxidant/antioxidant balance (Swamy et al. 2011). To induce oxidative stress in C2C12 myoblasts, the cells were incubated with H2O2 at a concentration of 100 μM. After H2O2 treatment, the viability of C2C12 myoblasts decreased to less than 60% compared to that in the control cells (Fig. 6). However, C2C12 myoblasts treated with HH and RG mixtures showed increased viability compared with that reported for the control cells. Notably, at a sample concentration of 500 μg/mL, cell viability increased steadily with increasing percentage of RG except at 100% RG, where only RG was present in the mixture.
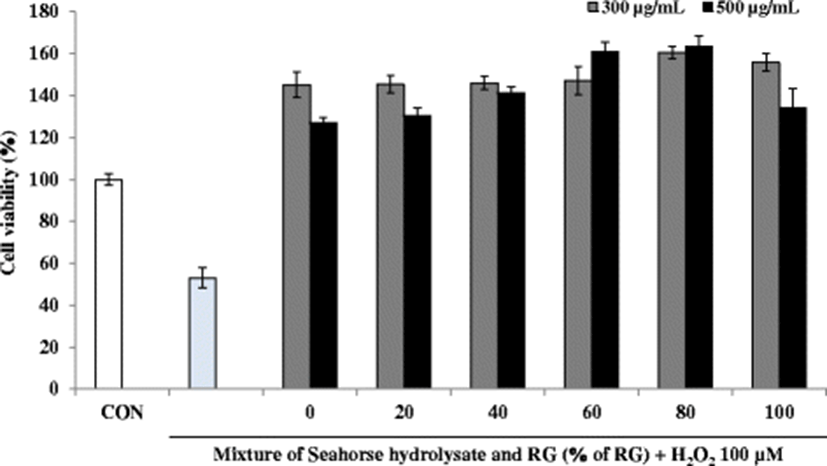
Energy expenditure during exercise leads to physical fatigue, which is mainly caused by energy consumption and deficiency. Catabolized fat and carbohydrates are considered the main sources of energy in the skeletal muscles during exercise, and glycogen is the predominant source of glycolysis for energy production. Therefore, glycogen storage directly affects exercise ability (Wu et al. 2013). The glycogen content of the H2O2-treated cells was lower than that of the control cells. However, in C2C12 myoblasts, the treatment with HH and RG mixtures increased the glycogen content to more than double as compared to the values reported for the control cells. In particular, RG60, RG80, and RG100 showed increased glycogen content at a sample concentration of 300 and 500 μg/mL (Fig. 7).
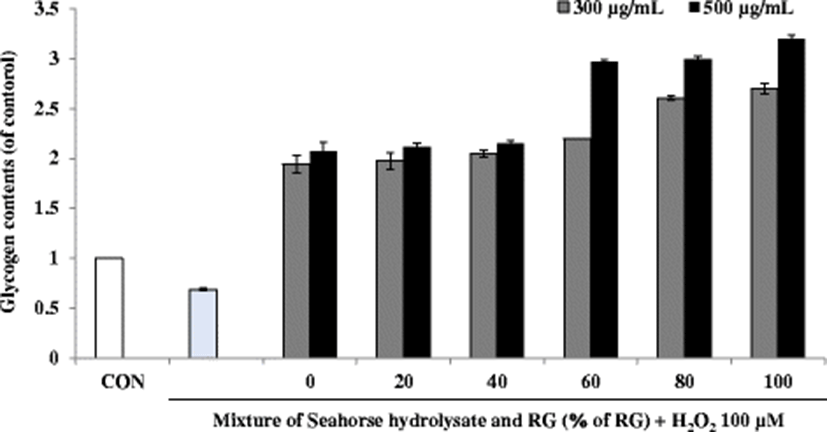
Muscular exercise causes rapid ATP consumption, and energy deficiency is a critical reason for physical fatigue. Therefore, compounds that promote ATP production could be candidates for alleviating physical fatigue. The skeletal muscle mainly catabolizes fat and carbohydrates as sources of energy during exercise (Nozawa et al. 2009). ATP content in the H2O2-treated cells was lower than that in the control cells (Fig. 8). Although HH and RG mixtures did not increase the ATP content, RG80 relatively increased the ATP content at 300 and 500 μg/mL concentrations of the mixture.
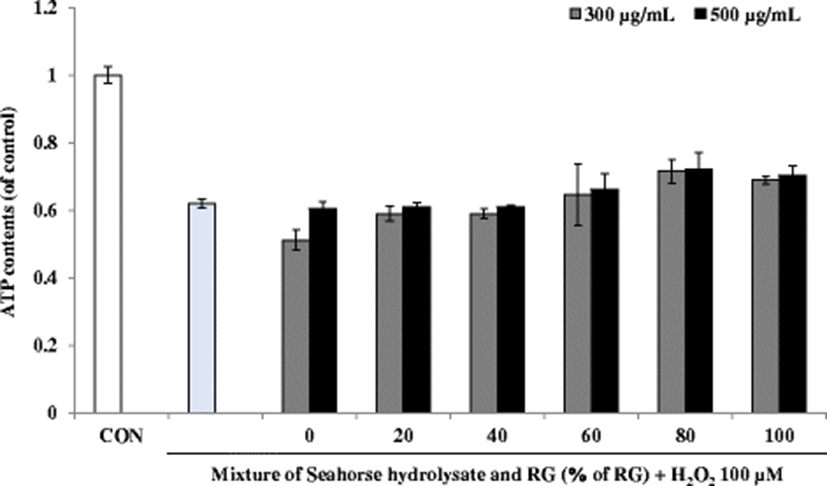
Exercise-induced oxidative stress can cause the increased muscle fatigability. Thus, antioxidants can decrease the oxidative stress and improve the physiological condition (You et al. 2011). Some reports showed that a loach peptide has not only antioxidant activities but also an anti-fatigue effect in mice (You et al. 2011). Actually, the peptide showing in vitro antioxidant activity possesses the in vivo anti-fatigue activity. The peptide acts as the scavenger for DPPH and hydroxyl radicals. Also, the anthocyanins of mulberry fruit have been assessed in vitro antioxidant activity and in vivo anti-fatigue activity (Jiang et al. 2013). These studies showed values of in vitro study to evaluate the potential anti-fatigue activity through in vivo study. In the present study, the mixtures of HH and RG acted as the antioxidant for hydrogen peroxide and showed the anti-fatigue activity on C2C12 myoblast. Furthermore, the mixtures have valuable needs to be investigated through in vivo animal study.
Conclusions
In this study, the effect of H. abdominalis on the muscles was investigated to scientifically verify its potential bioactivity. Also, the anti-fatigue activity of a mixture comprising HH and RG was evaluated to commercially utilize H. abdominalis in food industry. The treatment of HH to C2C12 myoblast induced the cell proliferation and glycogen contents. These results indicated that H. abdominalis had anti-fatigue activity on C2C12 myoblast. Moreover, the treatment of the mixture comprising HH and RG increased cell viability and the content of fatigue-related biomarkers such as glycogen and ATP contents. In particular, the 80% RG mixture showed an optimum effect on cell viability and ATP synthesis activity. These results indicated that HH had anti-fatigue activity at concentrations approved for use in food by the law in Korea. Especially, an 80% RG to HH mixture has the potential to ameliorate fatigue condition induced by oxidative stress by increasing the fatigue-related biochemical parameters such as glycogen and ATP contents in C2C12 myoblasts. Therefore, 80% RG to HH mixture can be used in food for ameliorating fatigue in Korea.