Introduction
Water temperature (WT), dissolved oxygen (DO) concentration, and photoperiod influence feed consumption, metabolic rate, and energy expenditure, and thus effect the growth of poikilothermic vertebrates, including fish (Lee et al., 2014; Liyanage et al., 2018; Shin et al., 2018). Therefore, the effects of these environmental factors on fish growth and metabolism warrant thorough investigation.
Many authors have demonstrated effects of various factors such as WT, salinity, photoperiod, fish size, feed amount, and stress on oxygen requirements of fish (Forsberg, 1994; Lee et al., 2014; Shin et al., 2018; Wei et al., 2019). In particular, in the case of aquaculture, DO content is considered an important factor for determining carrying capacity and production (Jørgensen et al., 1991; Lee et al., 2014; Shin et al., 2018). Moreover, DO content is a parameter used in the determination of density in aquaculture and live-fish transport, and to determine the amount of feed required (Buentello et al., 2000; Kim et al., 2011; Kim et al., 2018; Yang et al., 2017). To sustain optimal feeding activity and health of aquaculture fish, controlling DO in water is very important.
Change in WT is an environmental stress that directly affects the metabolism of rearing fish. Barton & Iwama (1991) suggested that a rapid change in WT resulted in changes to in vivo metabolism and hematology. A rapid drop in WT, for example due to the common occurrence of cold-water masses along the eastern coast of Korea in summer, affects the growth and survival of aquaculture fish (Chang et al., 2001). Additionally, an increase in WT, for example due to the release of heated effluent water from power plants and high WT during the summer season, also affects the health of fish in aquaculture. Power plants can release water from their cooling systems that is approximately 7°C higher than natural seawater (Nam et al., 2016). This heated effluent water causes rapid changes in WT in adjacent waters. Rearing fish cannot easily adapt to such temperature changes and the growth and survival of aquaculture fish can be directly influenced by these changes (Chang et al., 2001).
In the present study, the effects of WT changes on the oxygen consumption (OC) and hematological factors of olive flounder Paralichthys olivaceus, a potential aquaculture species, were determined. Such knowledge will be useful for evaluating current olive flounder aquaculture procedures and also for developing techniques to minimize stress during rearing. The results of the study can also be used as basic data to understand olive flounder death due to exposure to rapid temperature changes in aquaculture environments.
Materials and Methods
The olive flounder (mean length 19.1 ± 0.8 cm, mean weight 53.7 ± 6.3 g) used in this experiment were hatched in April 2019 at a flounder farm and subsequently transported to a mariculture facility at the Kunsan National University, Korea. Feed (extruded pellets) was supplied twice (at 09:00 h and 18:00 h) at the rate of about 3% body weight each day. Food was withheld from the fish for 24 h before each experiment. Thirty individual fish per chamber (2 × 1 × 0.5 m) were reared for experiments. The average rearing density of fish was 1.65 kg (m3)−1. The WT, salinity, and DO of the seawater during the period was 17.2°C–18.1°C, 33–35 psu, and 5.8–6.4 ppm, respectively.
Experimental fish were kept in a respiratory chamber (RC) for 12 h prior to recording DO in the inlet and outlet water (OW) in order to stabilize metabolic rate. They were not fed 24 h prior to or during the experiment. WT in each experiment was controlled with a circulating water bath (JS-WBP-170RP; Johnsam, Bucheon, Korea) and the fish were exposed to a cycle of 12-h light: 12-h dark (centered on 09:00) at an average light intensity of 1,032 lux measured at the experiment vessel surface. The physicochemical properties of the seawater used in the experiments were as follows: salinity, 35 psu; total nitrogen (T-N), 1.9–4.1 mg L−1; total kjeldahl nitrogen (TKN), 1.0–1.2 mg L−1; NH4+-N, 0.9–1.1 mg L−1; NO3−-N, 0.9–2.9 mg L−1; total phosphorus (T-P), 0.02–0.78 mg L−1; PO43–-P, 0.004–0.351; chemical oxygen demand using manganese (CODMn), 2.0–11.1 mg L−1.
The lowest temperature in this experiment was set to 11°C and the highest temperature to 29°C, considering that the seawater temperature in Korea varies from a minimum of 10°C to a maximum of approximately 27°C (Oh et al., 2011). In Exp. I, WT was increased from 20°C (control WT) to 29°C within 18 h (0.5°C h−1) and maintained at 29°C for 96 h. Then, WT was decreased from 29°C to 20°C within 18 h (0.5°C h−1) and maintained at 20°C for 24 h. In Exp. II, WT was decreased from 20°C (control WT) to 11°C within 18 h (0.5°C h−1) and maintained at 11°C for 96 h. Then, WT was increased from 11°C to 20°C within 18 h (0.5°C h−1) and maintained at 20°C for 24 h. The Exp. III maintained that the Exp. I and II was consecutively.
OC measurement systems consisted of closed water flowing systems with a RC and are shown in Fig. 1 (Chang et al., 2005). The RC (outer dimension 20 × 30 × 20 cm) had a hexagonal shape made of transparent PVC (thickness 10 mm), a water volume of 10.4 L, and a constant flowing rate throughout the experiments. Five fish were used for each experimental group, with a mean weight of 54.1 ± 2.4 g. One fish per chamber was used.
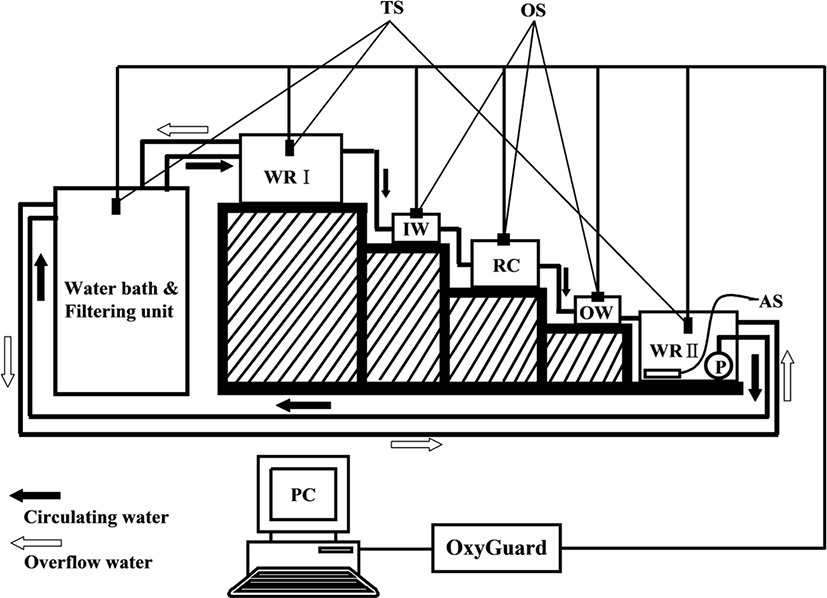
In water reservoir I (WRI), a constant water pressure was maintained by overflowing water (white arrow) when a certain water level was reached, which was then conducted through an inlet into the RC. The OW from the RC was transferred to water reservoir II (WRII) where it was saturated with oxygen. Water was then temperature controlled and cleaned in a water bath (WB) and filtering unit (FU), respectively, before reentering the RC.
The DO content of the inlet water (IW) in each experiment was maintained above 5.7 mg O2 L−1 at 20°C. The DO content of the IW and OW in each experiment was measured every 10 min automatically through each oxygen sensor (OS) with a multichannel monitoring system for DO and other parameters (OxyGuard 6; OxyGuard International A/S, Farum, Denmark) and the obtained data were input to a personal computer (PC).
In the experiment of OC to WT, the WT in the RC was slowly increased from 20°C to the target temperature at a rate of 0.5°C h−1 to minimize potential thermal shock to experimental fish. In all experiments, fluorescent lights were used during the light period, and during the dark period the whole RC containing experimental fish was covered with three layers of black polyethylene film to exclude light from outside. To account for possible bacterial consumption of oxygen in the system, a blank trial without fish was run after each experiment. Corrections for possible bacterial consumption were not necessary as there was negligible change in oxygen concentration over the hour.
In addition to measuring OC, the behavior of fish was observed during light and dark periods; swimming and resting, position in the water, and breathing frequency of each fish as well as attacks and escapes were recorded or counted. The ventilation rates were counted by opercular cover movements (Ingram & WaresII, 1979). The opercular cover movements were counted in 1-min intervals and expressed as the average rate calculated from 10 records of each fish.
The OC of fish was calculated with the following formula: OC = (DOin – DOout) × F/W (Chang et al., 2005), where OC is the OC expressed as milligrams of oxygen per hour per kilogram of fish (i.e., mg O2 kg−1 h−1); DOin and DOout are the DO (mg O2 L−1) in the inlet and OW, respectively; F is the water flow (L min−1); and W is fish biomass (kg).
The blood of olive flounders was sampled from different tanks before and after the experiments. Blood samples were collected from the caudal blood vessel complex using heparinized syringes within 1 min without anesthesia.
Hematocrit, red blood cells, and hemoglobin were analyzed immediately using an automatic blood analyzer (BC-2800VET, Mindray, Nanshan, China). Blood samples were kept in 2-mL vacuum containers treated with sodium fluoride/potassium oxalate and in 1.5-mL polypropylene microcentrifuge tubes held on ice for less than 5 min before centrifugation at 5,600 g for 5 min. Plasma was then collected and stored in a deep freezer (CLN-500 UW Nihon Freezer; Nihon Freezer, Tokyo, Japan) at –70°C until analysis. Plasma cortisol concentrations were determined using a cortisol analysis kit (DRI-CHEM, Fuji Film, Tokyo, Japan). Glucose, lactic acid, aspartate aminotransferase (AST), alanine aminotransferase (ALT), Na+, K+, Cl−, and total protein were analyzed using an automatic chemistry analyzer (DRI-CHEM NX500i, Fuji Film, Japan). Osmolality was determined using a micro-osmometer (Fiske 210; Fiske, London, UK).
The experiments were performed in triplicate and results are reported as means ± SD (n = 5) unless otherwise stated. Data were analyzed by one-way ANOVA with the SPSS statistical package (SPSS, Chicago, IL, USA). Means were separated using Duncan’s multiple range test and were considered significantly different at p < 0.05.
Results
Changes in the OC and respiration number in Exps. I and II are shown in Fig. 2 and Table 1. In Exp. I, the average OC when WT was maintained at 20°C for 24 h was 116.7 mg O2 kg−1 h−1, and the respiration number per minute was 80.0 ± 17.6. The average OC increased to 227.5 mg O2 kg−1 h−1 when WT was increased from 20°C to 29°C. The average OC during the 4 d at 29°C was 317.5 mg O2 kg−1 h−1, which was higher than the average OC when WT was increased. The respiration number per minute at 29°C was 112.0 ± 15.1, which was higher than that at 20°C. When WT was maintained at 20°C for 24 h, the average OC and respiration number per minute decreased to 130.3 mg O2 kg−1 h−1 and 91.1 ± 15.5, respectively, and the respiration number was higher at the end of the experiment than at the start of the experiment.
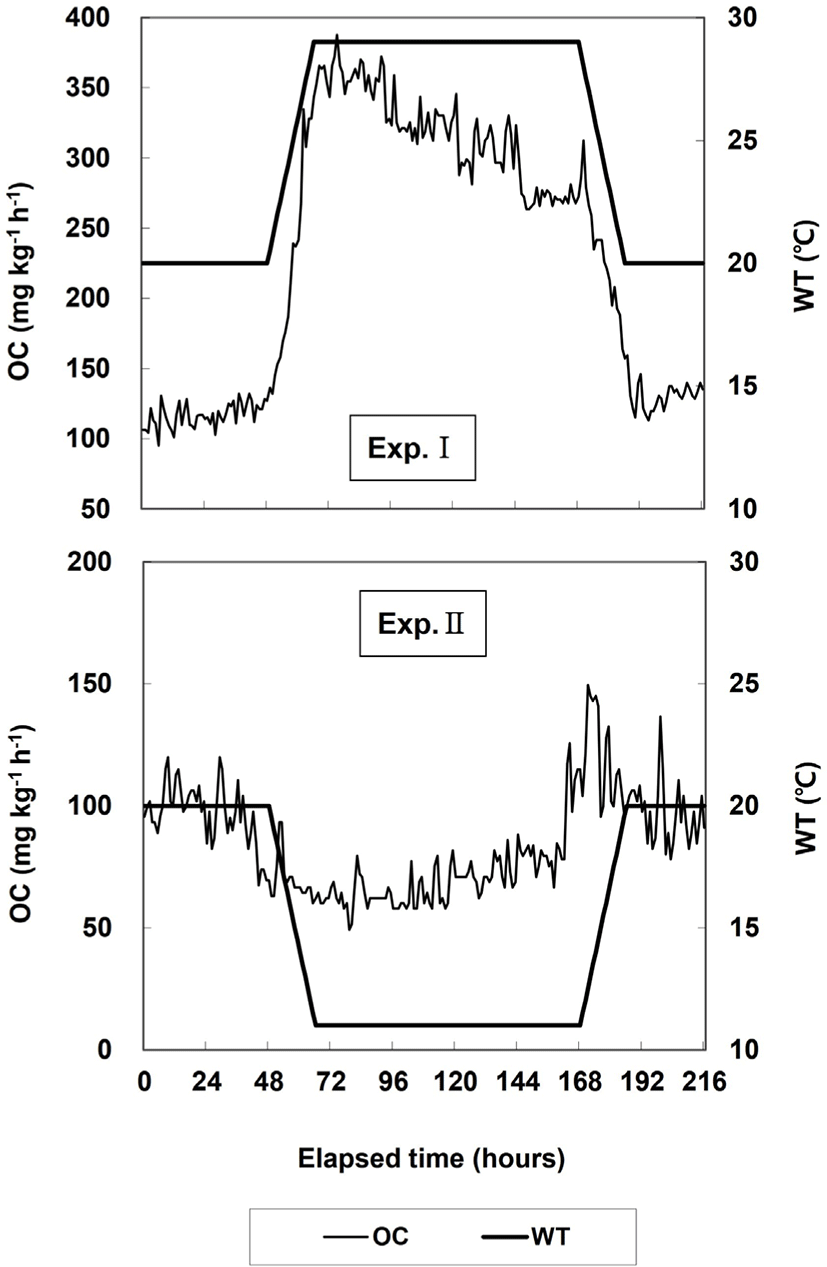
In Exp. II, when WT was maintained at 20°C for 48 h, the average OC was 96.5 mg O2 kg−1 h−1 and the respiration number per minute was 90.0 ± 13.7. When WT was reduced to 11°C, the average OC decreased to 69.6 mg O2 kg−1 h−1. When WT was maintained at 11°C for 4 d, the average OC was 71.3 mg O2 kg−1 h−1, which was similar to the average OC when WT was reduced. The respiration number per minute at 29°C decreased to 72.0 ± 22.2 and was lower than the respiration number at 20°C. When WT was increased to 20°C, the average OC increased to 118.7 mg O2 kg−1 h−1, but when WT was maintained at 20°C for 24 h it was 96.7 mg O2 kg−1 h−1, which was similar to the OC value at the start of the experiment. The respiration number per minute at 20°C decreased to 66.1 ± 21.3, which was the lowest value in Exp. II.
The changes in OC in Exp. III are shown in Fig. 3. In Exp. III, when WT was maintained at 20°C for 48 h, the average OC was 117.1 mg O2 kg−1 h−1 and the respiration number per minute was 88.0 ± 36.2. During the 3 d at 29°C, the respiration number per minute and the average value of OC were 116.3 ± 42.8 and 226.1 mg O2 kg−1 h−1, respectively, which were higher than those before WT was increased. OC and the respiration number decreased to 108.4 mg O2 kg−1 h−1 and 91.1 ± 31.6, respectively, during the 3 d when WT was maintained at 20°C. When WT was reduced to and maintained at 11°C for 3 d, the average OC and respiration number per minute decreased to 54.4 mg O2 kg−1 h−1 and 60.0 ± 29.8, respectively. The respiration number at 11°C was the lowest value in all of the experimental groups, including in Exps. I and II. When WT was increased to and maintained at 20°C for 48 h, the respiration number per minute and average OC increased to 87.1 ± 46.1 and 110.2 mg O2 kg−1 h−1, respectively.
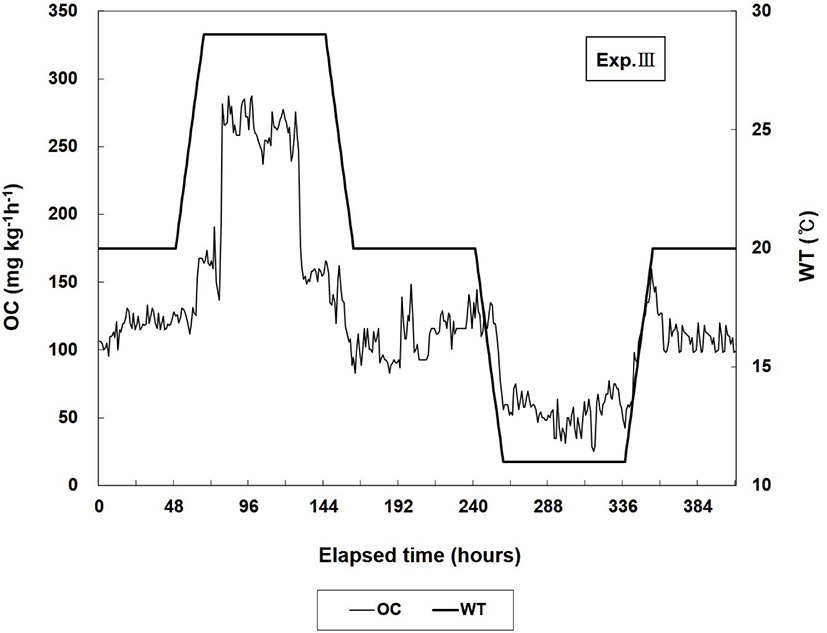
The hematological characteristics in Exps. I, II, and III are shown in Table 2. The hematocrit values in Exps. I and III differed significantly between the end of the experiments (after WT was changed) and the beginning of the experiments (before WT was changed; p < 0.05). In Exp. II, the hematocrit values did not differ significantly between the beginning and the end of the experiment (p > 0.05). Furthermore, RBC and hemoglobin did not differ significantly between the beginning and end of the experiment in Exps. I, II, or III (p > 0.05). The cortisol concentration did not differ in Exp. II (p > 0.05), but increased from 4.8 ± 0.9 ng mL−1 at the start of the experiment to 8.2 ± 1.4 ng mL−1 at the end of the experiment in Exp. I. In Exp. III, the cortisol concentration increased significantly from 6.0 ± 0.8 ng mL−1 at the beginning of the experiment to 15.0 ± 1.1 ng mL−1 at the end of the experiment. In Exps. I and III, the glucose concentrations showed similar trends to the hematocrit and cortisol concentrations, and the glucose concentration was higher at the end of the experiment than at the beginning. Cl−, osmolality, AST, and ALT differed significantly between the start and end of the experiment in Exp. II (p < 0.05).
Discussion
WT is a major environmental factor that affects the metabolic rates of fish, with direct effects on their food intake, ammonia excretion, OC, growth, and survival (Barkai & Griffiths, 1988; Cho et al., 2015; Hahn, 1989; Jaxion-Harm & Ladich, 2014; Kim et al., 2018; Liyanage et al., 2018; Lyon, 1995; Peck, 1989; Shin et al., 2018). Temperature tolerance and tolerance to environmental changes are widespread adaptations in fish, and each fish species has an optimal WT for growth (Shamseldin et al., 1997).
Cho et al. (2015) reported the effects of WT changes on the hematological responses and plasma cortisol concentrations in growing red spotted grouper, Epinephelus akaara. The hematocrit and glucose concentrations for the red spotted grouper were significantly higher at 15°C than at 20°C or 25°C. The hemoglobin value also increased at 15°C, but not significantly. The plasma glucose concentrations of the red spotted grouper did not correlate with the observed plasma cortisol response. There were no differences in cortisol concentrations among the temperature groups (Cho et al., 2015). Jaxion-Harm & Ladich (2014) reported the effects of WT changes on plasma cortisol concentrations in common carp. Cortisol levels of common carp at the repeat 20°C trial were significantly lower than during the first 20°C trial (Jaxion-Harm & Ladich, 2014). In addition, cortisol concentrations of common carp were significantly higher at 20°C than at 14°C (Jaxion-Harm & Ladich, 2014). In our experiment, some of the results were consistent with those of Jaxion-Harm & Ladich (2014) and Cho et al. (2015), but others were not. The hemoglobin values of the olive flounder did not change, but the glucose concentration increased in Exp. II. The cortisol concentrations in Exp. II decreased with decreasing WT. However, the cortisol and glucose concentrations did not change significantly between the beginning and the end of the experiment. In Exps. I and III, the hematocrit, cortisol, and glucose concentrations of the olive flounder increased with increasing WT. Cortisol and glucose concentrations are usually indicators of stress in fish (Cho et al., 2015). High cortisol and glucose concentrations are indicative of retarded metabolism and are also indices of sublethal stress (Barton & Iwama, 1991; Best et al., 2001; Cho et al., 2015). Therefore, Exp. III (increasing and decreasing WT) probably imposed a more stressful environment on the olive flounder than did Exp. I (increasing WT) or Exp. II (decreasing WT).
The effects of high temperatures on olive flounder were observed in Exp. I. It can be seen from this study that olive flounder increased their oxygen demand and respiration volume and experienced significant stress when exposed to an environment of 30°C. This suggests that olive flounder can be stressed when exposed to high temperature environments, which could negatively affect production and mortality. The physiological changes in olive flounder when exposed to a low temperature environment were observed in Exp. II. In this study, when exposed to a low temperature environment, olive flounder decreased OC, respiratory rate, and osmotic pressure in the body. Prolonged exposure to a low temperature environment does not cause a stress response due to metabolic decline but may lead to changes in osmotic pressure in the body. The physiological changes in olive flounder under rapid temperature fluctuations were observed in Exp. III. In this study, a sudden change in temperature showed an extreme stress response accompanied by a change in OC. This could lead to a decrease in productivity or even mass death.
Kim et al. (1995) reported that within the range of optimal WT, the OC of fish generally increased in direct proportion to the increase in WT (Oh et al., 2007), and the results of our experiment are consistent with this. Kim et al. (1995) reported OC values of 75.7 and 698.1 mg O2 kg−1 h−1 at 15.2°C and 24°C, respectively, after black rockfish were starved (average weight: 3.45 g), and OC increased with increasing temperature. Exps. I and III in our study confirmed that OC increases with increasing WT. Kim et al. (1995) also suggested that the OC of the black rockfish (mean bodyweight: 1.4 g) has the following linear relationship when expressed as a function of WT: OC = 75.146WT − 947.937. In our study, the OC of the olive flounder also increased directly with increasing WT. Prosser & Brown (1961) stated that the standard metabolism of fish increases continuously with temperature up to the lethal temperature, suggesting a rate change of 2.5 times per 10°C within the physiological temperature range (Kim et al., 1995). Therefore, the metabolism of the olive flounder increased in direct proportion to the increase in WT.
In this study, the OC of the olive flounder was lower than those of other species reported in a previous study. Kim et al. (1995) found that OC changes in response to WT differed among species. The OC of benthic fish, such as the olive flounder, was the lowest among six species tested (olive flounder; black rockfish; black seabream, Acanthopagrus schlegeli; red seabream, Pagrus major; sea bass, Lateolabrax japonicas; tiger puffer, Takifugu rubripes). Some active swimmers, such as the tiger puffer and the black rockfish, consume a lot more DO than the olive flounder. When engaged in vigorous activity, the red seabream and black seabream consumed the most DO (Kim et al., 1995). Therefore, Kim et al. (1995) reported that actively swimming species consume much more DO than benthic settling species.
In our study, OC was measured after 24 h of starvation in all the experimental groups. Therefore, the OC for feed intake and digestion should be regarded as extremely suppressed at that time. The gastric evacuation time after feeding in the olive flounder has not been reported. The gastric evacuation time of another inactive fish, the black rockfish (S. schlegeli), is 21 h, and its OC is approximately 250 mg O2 kg−1 h−1, whereas in our study, that of the olive flounder was approximately 100 mg O2 kg−1 h−1 at 20°C (Kim & Chin, 1995; Kim et al., 1995; Oh et al., 2007). The gastric evacuation time of the olive flounder could be longer than that of the black rockfish because the olive flounder is less active than the black rockfish. Therefore, the difference in OC according to the gastric evacuation time between active and inactive fish warrants further investigation.
Oh & Noh (2006) reported that the circadian rhythm of OC in the dark-banded rockfish, S. inermis, showed that OC increased in the light phase and decreased in the dark phase. Thus, the photoperiod affects OC in the dark-banded rockfish. In our study, the OC of the olive flounder did not change in response to the photoperiod during the experimental period. The olive flounder may not be significantly affected by the photoperiod as compared with other fish because it is a benthic fish.
In this study, OC tended to increase with increasing WT, and decreased with decreasing WT. Therefore, OC increased in proportion to the increase in WT, which agrees well with the principle of the thermal quotient (Q10 value) as a metabolic index. Our results will be useful to evaluate the current aquaculture procedures used for the olive flounder and for the development of techniques to minimize stress in aquaculture farms.