Introduction
The industrial revolution has led to a significant and rapid increase in the emissions of carbon dioxide into the atmosphere. This rise in carbon emissions is mostly attributed to anthropogenic activity, with fossil fuels and land use conversion, respectively accounting for 90% and 10% of these CO2 emissions (Friedlingstein et al., 2023). Prior to the industrial revolution, the level of carbon dioxide in the atmosphere was approximately 278 parts per million (Betts, 2021). Nevertheless, this level has steadily increased over the past decades. The average global atmospheric concentration of CO2 in 2022 was 417.1 ± 0.1 ppm. On March 10, 2024, the concentration of atmospheric CO2 was 421.56 ppm, representing a 51.6% increased relative to the pre-industrialization era (Global Monitoring Laboratory, 2024).
Increasingly higher levels of atmospheric CO2 are being absorbed by the world’s oceans at an accelerating rate. Since the onset of worldwide industrialization, the ocean has absorbed approximately 20%–30% of all anthropogenic carbon dioxide (CO2) emissions (Jiang et al., 2023). Over the past few decades, the oceans have absorbed approximately 2 billion metric tons of CO2 out of the total annual carbon emissions of 7 billion metric tons released into the atmosphere by all nations worldwide. It is projected that by 2100, the seas will absorb around 5 billion metric tons of CO2 annually (Upton & Folger, 2013).
Ocean acidification is the process by which the pH of seawater falls due to the ocean’s increasing absorption of anthropogenic CO2 from atmosphere (Upton & Folger, 2013). Oceanic CO2 absorption triggers a chemical reaction whereby carbon dioxide and water produce carbonic acid (H2CO3). In turn, carbonic acid undergoes dissociation, producing hydrogen ions (H+) and bicarbonate ions (HCO3–). Bicarbonate ions (HCO3–) then dissociate, resulting in the formation of H+ and CO32– ions. This is the primary factor responsible for the decrease in oceanic pH. Crucially, hydrogen ions (H+) in water can also react with carbonate ions (CO32–) to produce bicarbonate ions (HCO3–), leading to a reduction in the concentration of CO32– ions, which are essential for the formation of shells and bones in marine organisms.
Since the beginning of the industrial revolution, seawater pH levels have experienced an overall reduction of pH 0.1 units (Melendez & Salisbury, 2017), with the average pH of oceanic surface waters falling from 8.2 to 8.1 (Logan, 2010). This 0.1 unit decline has resulted in a 30% increase in seawater acidity over the course of more than 200 years (Laffoley et al., 2017), and oceanic pH levels are expected to continue decreasing as atmospheric CO2 absorption levels rise. Melendez & Salisbury (2017) have predicted a 120% increase in the acidity of seawater by the year 2060. Furthermore, Basso et al. (2015) projected that the pH of seawater will fall by approximately 0.3–0.4 units by 2100 and 0.7–0.8 units by 2300.
Ocean acidification poses a serious threat to marine organism, particularly calcifying animals such as abalone, as they rely on calcium carbonate (CaCO3) for shell formation (Wessel et al., 2018). Several studies have already documented the impact of pH fluctuations on various aspects of abalone biology such as hatching rate (Li et al., 2013), larval development (Tahil & Dy, 2016), larval settlement (Tahil & Dy, 2015), immunity, stress response (Kim et al., 2023), growth, and shell thickness (Cummings et al., 2019). Abalone, a globally distributed gastropod, is a highly valuable seafood product. The high demand and price of abalone have driven the global expansion of abalone aquaculture, with this sector experiencing significant growth in recent years. However, decreased pH levels could severely undermine the sustainability of abalone aquaculture, thereby hindering the advancement of abalone production (Tahil & Dy, 2016).
This review aims to comprehensively examine the scientific literature on the impact of ocean acidification on abalone, focusing on early life stages, juvenile and adult development, shell thickness, and physiology. Understanding the impact of pH on abalone will provide a valuable basis for the development of farming procedures to reduce stress, enhance productivity, and promote abalone health and quality (Morash & Alter, 2016).
Economical Values of Abalone
Abalone (Haliotis spp.) is a highly valuable commodity due to its premium price, high nutritional value, and unique flavor. Abalone products can be consumed in various ways, including cooked, raw, chilled, and canned (Taridala et al., 2020). Abalone has also been shown to contain a number of bioactive compounds, including phenolic compounds, glycogen, carbohydrates, protein, amino acids (Mohammadi et al., 2022), fatty acids (Suleria et al., 2017), and minerals (Latuihamallo et al., 2019), all of which offer health-promoting effects beyond those of basic nutrition. Nutritional analyses revealed that 100 g of the edible portion of abalone contained 20 grams of high-quality protein (Shi et al., 2020). According to Mulvaney et al. (2015), 100 g of abalone also contains 94 mg of n-3 long-chain polyunsaturated fatty acids (LC PUFA), which is higher than the 70, 26, and 50 mg of PUFA found in beef, pork, and chicken, respectively. Furthermore, abalone possesses anti-microbial, anti-thrombotic, anti-cancer activities, and anti-inflammatory properties (Suleria et al., 2017).
Abalone production has steadily increased due to strong market demand over the past decade. The total abalone production from aquaculture and capture fisheries has increased significantly, from 14,599.00 tons in 2001 to 253,111.71 tons in 2021 (Fig. 1). In the past, worldwide abalone production derived mainly from fishing. However, aquaculture now makes up the overwhelming majority of global abalone production (Hernández-Casas et al., 2023). As of 2021, aquaculture accounted for more than 97% of the total global abalone production, achieving a total output 245,777.34 tons (Fig. 1) and an estimated value of $2.59 billion (Fig. 2). In contrast, production of captured abalone decreased considerably from 11,529 tons in 2001 to 7,334.37 tons (Fig. 1). The depletion of abalone populations in various Southeast Asian countries, such as Malaysia, Thailand, Indonesia, and the Philippines, suggests that the natural supplies of abalone have reached a critical threshold (Sososutiksno & Gasperz, 2017). In turn, this reduction in abalone fishing yields has been attributed to a number of factors, including the degradation of marine ecosystems, diseases, illegal fishing, overexploitation, and rising operational costs (Hernández-Casas et al., 2023).
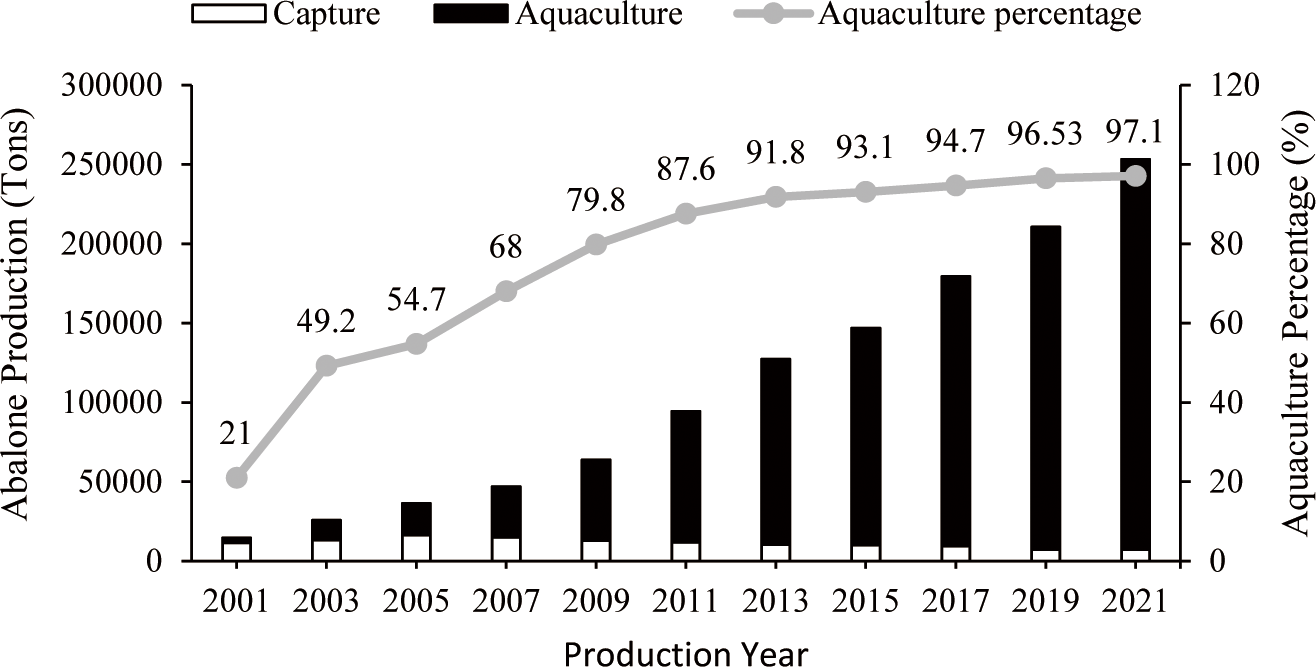
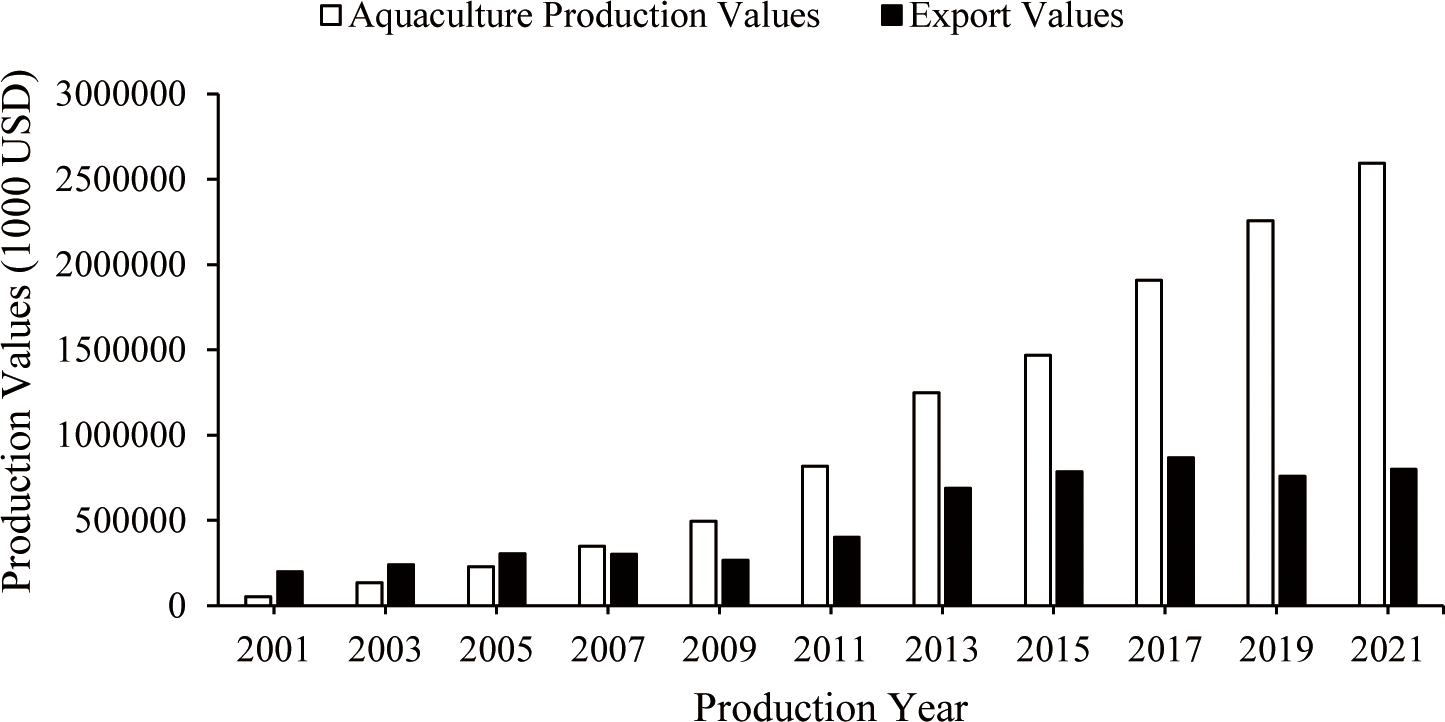
In 2021, China dominated the abalone aquaculture industry, producing 217,831 tons valued at $1.85 billion (FAO, 2024). Chinese abalone farms are mostly located in the provinces of Fujian and Guangdong, although a significant proportion of abalone seed production takes place between the Shandong Peninsula and Dalian, in the Northern Province of Liaoning (Cook, 2023). According to FAO (2024), South Korea ranks second with a production of 23,199.49 tons valued at $606 million (Table 1). Most of the production in Korea takes place in Wando County, located in the province of South Jeolla. Cage culture covers a total area of 3,095 hectares across 2,549 abalone farms in Korea (Cook, 2023). Chile, South Africa, New Zealand, Australia, and the United States have also engaged in abalone cultivation (FAO, 2024). However, in a few countries, including Australia, Japan, Mexico, and New Zealand, abalone capture fisheries remains the dominant source of abalone production. Among these countries, Australia is the largest producer of captured abalone, with a total catch of 2,352.13 tons (FAO, 2024).
Adapted from FAO (2024) with public domain.
The global abalone export value has also increased, from over US$200 million in 2001 to US$800 million in 2021, clearly demonstrating the growing demand for abalone (Fig. 2). China ranks first in terms of abalone export value with a total value of US$437 million (FAO, 2024), followed by Australia with a value of US$120 million, South Africa with US$61 million, and South Korea ranks fourth with a value of US$54 million (Table 2; FAO, 2024). The price of abalone in different nations varies according to the species, size, location of origin, and quality of the abalone meat. Abalone with a shell size of 40 mm sells for US$10 per kilogram in the Chinese seafood market, whereas larger abalone sells for US$100 per kg. In 2023, the wholesale price of abalone in Korea ranged from US$26.4 to US$28.7 per kg, with retail buyers paying far higher costs (Cook, 2023). Future demand for abalone is expected to increase, which is likely to result in an ongoing expansion of abalone production. Therefore, it is crucial for farmers to reduce production costs and enhance production efficiency.
Adapted from FAO (2024) with public domain.
Ecological Values of Abalone
Abalone can significantly influence both the structure of ecological communities and the functioning of ecosystems through several means, such as their role as grazers and the provision of microhabitats for benthic organisms (Zeeman et al., 2013). Additionally, these gastropods are considered valuable environmental bioindicators (Liao et al., 2004). As herbivores, abalone play a significant role in controlling the population of algae and other invertebrates that compete for the same food (Zeeman et al., 2012). Abalone and sea urchins are primary competitors, as they both consume kelp. Assuming that abalone populations were at their typical levels, they would effectively control the sea urchin populations and prevent them from excessively feeding on kelp. Sea urchins and abalone are the predominant herbivorous animals in the kelp forests of Australia, South Africa, and the North Pacific and they exhibit significant overlap in their food preferences and habitat utilization (Luo et al., 2023). Additionally, post-larval abalone has been shown to compete for food resources with the small Sea snail (Homalopoma amussitatum) (Won et al., 2011).
According to Zeeman et al. (2013), South African Abalone (Haliotis midae) shells serve as a microhabitat for benthic organisms, including coralline algae such as Titanoderma polycephalum, Melobesioid alga (Mesophyllum engelhartii), as well as members of the genus Spongites. Due to its ability to thrive in abundant numbers, its flat shell with uneven ridges, and long lifespan, this abalone species serves as a habitat for a wide range of organisms. Moreover, given the scarcity of hard surface areas in marine ecosystems, gastropod shells serve as a significant secondary substrate for colonization. Interestingly, however, the populations inhabiting the surface of abalone shells are distinct from the species residing on the neighboring rock. Hence, the excessive fishing of abalone can have a significant impact on the entire ecosystem through both top-down (e.g., predation) and bottom-up (e.g., competition) interactions. This can result in a trophic cascade within the ecosystem, potentially leading to a shift to an alternative stable state (Won et al., 2011).
An effective bioindicator should be immobile, plentiful, long-lived, easily collectible, capable of accumulating pollutants, and providing appropriate tissue for contaminant analysis (Liao et al., 2004). Abalone fulfills all of these criteria, making them excellent bioindicators for marine environments. Previous study have reported that abalone are able to accumulate zinc (Liao et al., 2004), cadmium, copper, and iron (Skinner et al., 2004). The shell (Liao et al., 2004) and foot muscle (Skinner et al., 2004) of abalone can thus be utilized for detecting the accumulation of heavy metals. Abalone can serve as a bioindicator and effectively remove heavy metals from aquatic food webs (Liao et al., 2004).
Impact of Ocean Acidification on Abalone
Ocean acidification changes the saturation of calcium carbonate in aquatic environments, thereby affecting marine shelled mollusks such as abalone, as well as other organisms that require calcium carbonate to form their skeletons and other similar anatomical structure (e.g., coral spicules). Alterations in pH also leads to acidosis, which hampers several physiological functions (Kavousi et al., 2022). Mollusk larvae are often more vulnerable to pH stress compared to adult mollusks due to their lack of specialized ion-regulatory epithelia necessary for maintaining acid-base balance (Parker et al., 2013). During the early stages of development, a lower pH can decrease fertilization rates, delay embryonic development, reduce hatching rate, larval length, and shell growth rates, and increase the malformation rate (Li et al., 2013). A summary of the impacts of ocean acidification on the early development of abalone (Haliotis spp.) is presented in Table 3.
Species | CO2/pH range | Experiment duration | Measured | Findings | References |
---|---|---|---|---|---|
Haliotis tuberculata | 8.2, 7.9, 7.8, and 7.6 | 15 min | Fertilization rate | NS | Byrne et al., 2010 |
Haliotis coccoradiata | 8.2, 7.9, 7.8, and 7.6 | 15 min | Fertilization rate | NS | |
H. coccoradiata | 7.8 and 7.6 | 21 h | Shelled larvae | Decrease under low pH | Byrne et al., 2011 |
Haliotis kamtschatkana | 400, 800, and 1,800 ppm (pH 8.3, 8.07, 7.81) | Total duration 8 d | Number of larvae | Decrease under low pH | Crim et al., 2011 |
Normal shell | |||||
Shell length | |||||
Settlement rate | NS | ||||
Haliotis discus hannai | 450, 650, 850, and 1,050 µatm (pH 7.96, 7.83, 7.79, 7.73) | 15 h | Fertilization rate | NS | Kimura et al., 2011 |
Hatching rate | Decrease at 650–1,050 µatm | ||||
75 h | Mortality | NS | |||
Malformation rate | NS | ||||
Shell length | Decrease at 850–1,050 µatm | ||||
H. discus hannai | 500, 1,100, 1,650, and 2,150 µatm (pH 7.94, 7.68, 7.54, 7.43) | 15 h | Fertilization rate | Decrease at 1,650–2,150 µatm | Kimura et al., 2011 |
Hatching rate | Decrease at 1,650–2,150 µatm | ||||
H. discus hannai | 500, 1,100, 1,650, and 2,150 µatm (pH 7.94, 7.68, 7.54, 7.43) | 75 h | Mortality | NS | Kimura et al., 2011 |
Malformation rate | Increase at 1,100–2,150 µatm | ||||
Shell length | Shorter at 1,650–2,150 µatm | ||||
H. discus hannai | 8.2, 7.9, 7.6, and 7.3 | 15 h | Hatching time | Longer at pH 7.6–7.3 | Li et al., 2013 |
Hatching rate | Decrease at pH 7.3 | ||||
60 h | Malformation rate | Increase at 7.6 and 7.3 | |||
48 h | Metamorphosis rate | Decrease at pH 7.3 | |||
Shell length | Shorter at 7.6 and 7.3 | ||||
H. discus hannai | 400, 800, 1,500, 2,000, and 3,000 ppm (8.15, 7.94, 7.71, 7.61, 7.43) | 30 min | Fertilization rate | Decrease at 3,000 ppm | Guo et al., 2015 |
8 h | Hatching rate | Decrease at 1,500, 2,000, 3,000 ppm | |||
24 and 48 h | Shell length | Shorter at 800 ppm | |||
Haliotis diversicolor | 400, 800, 1,500, 2,000, and 3,000 ppm (8.15, 7.94, 7.71, 7.61, 7.43) | 30 min | Fertilization rate | Decrease at 2,000–3,000 ppm | |
8 h | Hatching rate | Decrease at 1,500, 2,000, 3,000 ppm | |||
24 and 48 h | Shell length | Shorter at 800 ppm | |||
Haliotis asinina | 7.9–58.03, 7.63–7.89, 7.46–7.70, and 7.24–7.52 | 15 d | Settlement rate | Decrease at low pH | Tahil & Dy, 2015 |
H. asinina | 7.95–7.99, 7.76–7.80, 7.58–7.62, and 7.37–7.41 | 6.4 h | Hatching rate | Decrease at pH 7.6 and 7.4 | Tahil & Dy, 2016 |
Normal hatch trocophore | |||||
Haliotis rufescens | 7.1, 7.3, 7.5, 7.7, 7.9 | 600 s | Survival rate | Decrease under low pH | Boch et al., 2017 |
H. asinina | 8.15, 7.85, and 7.65 | 24 h | Shell length | Decrease under low pH | Santander & Sayno, 2018 |
Malformation rate | Increase at low pH | ||||
Haliotis tuberculata | 8.0, 7.7, and 7.6 | Total duration 5 d (sampling at 20, 30, 48, 96 hpf) | Larval viability | Decrease under low pH | Wessel et al., 2018 |
The percentage of shelled larvae | Lower at pH 7.6 | ||||
Shell calcification | Decrease at ph 7.6 | ||||
Haliotis iris | 8.05 and 7.65 | Settlement rate | NS | Espinel-Velasco et al., 2021 | |
Haliotis tuberculata | 8.0 and 7.7 | Total duration 96 h (sampling at 24, 72, 96 hpf) | Hatching rate | NS | Kavousi et al., 2022 |
Swimming rate | NS | ||||
Shell formation | Decrease at low pH for 24 and 72 hpf larvae | ||||
H. tuberculata | 8.0 and 7.7 | Total duration 96 h (sampling at 24, 72, 96 hpf) | Tissue organogenesis | Decrease at low pH for 24 and 72 hpf larvae | Kavousi et al., 2022 |
Shell lenght | Shorter at low pH for 72 hpf larvae | ||||
Shell calcification | Decrease at low pH for 72 hpf larvae | ||||
Larval settlement | NS | ||||
H. tuberculata | 8.0 and 7.7 | Larval swimming behaviour | NS | Kavousi et al., 2022 | |
Haliotis rufescens | 8.0, 7.8, and 7.6 | 48 and 108 h | Veliger maturation | Decrease at pH 7.6 | Gómez-Reyes et al., 2023 |
Short term and long term | Settlement rate | Decrease under low pH for short term experiment | |||
Haliotis discus hannai | 8.2, 7.9, 7.6, 7.3, and 7.0 | 30 hpf | Hatching rate | NS | Pratiwi et al., 2024 |
Larvae length | Decrease at pH 7.0 | ||||
Malformation rate | Increase at pH 7.0 and 7.3 |
Kimura et al. (2011) performed two experiments to examine the effects of ocean acidification on the fertilization success of Ezo abalone (Haliotis discus hannai). The initial experiment involved exposing seawater to CO2 partial pressures (pCO2) of 450, 650, 850, and 1,050 µatm, resulting in seawater pH values of 7.96, 7.83, 7.79, and 7.73, respectively. In this first experiment, the 1,050 µatm treatment resulted in the lowest rate of fertilization. The second experiment involved setting pCO2 levels at 500, 1,100, 1,650, and 2,150 µatm, which resulted in pH values of 7.94, 7.68, 7.54, and 7.43, respectively. In this case, a pCO2 level of < 1,100 µatm did not have any impact on the fertilization rate of abalone. However, a pCO2 of 1,650 µatm (pH 7.54) resulted in the lowest fertilization rate, followed by treatment with pCO2 of 2,150 µatm (pH 7.43). These data indicates that the optimal pCO2 range for ezo abalone is between 1,000 and 1,600 μatm. This outcome is comparable to that of Red abalone (Haliotis rufescens), which exhibited a drop in fertilization rate when exposed to a pH ranging from 7.95 to 7.2. Particularly, a pH below 7.55 under 9°C and 13°C seawater temperatures resulted in an apparent reduction in fertilization rate (Boch et al., 2017). According to Byrne et al. (2010), the fertilization rate of Reddish-rayed abalone (Haliotis coccoradiata) did not vary significantly across a pH range of 8.2 to 7.6. Moreover, Boch et al. (2017) indicated that lower fertilization rates may be due to the potential impact of lower pH on sperm kinematic and/or swimming behavior. Shi et al. (2017) demonstrated that excessive CO2 absorption notably impairs sperm motility in Blood clams (Tegillarca granosa). The ocean absorbs excess carbon dioxide in the atmosphere, increasing the quantity of hydrogen ions. These ions penetrate into the cells, causing intracellular acidosis and modifying intracellular pH. A decrease in intracellular pH can disrupt the sperm activation, sperm chemotactic behavior, and flagellar motility (Shi et al., 2017). Lower pH levels may diminish sperm mitochondrial membrane potential (Schlegel et al., 2015), limiting sperm flagellar motility (Shi et al., 2017), and impairing the activity of dynein ATPase, a protein involved in sperm movement (Boulais et al., 2018). Similar to acidic pH conditions, lower sperm concentrations have also been linked to reduced fertilization rates (Parker et al., 2013).
Acidification also negatively impacts the hatching rate and viability of fertilized eggs. In the Donkey ear abalone (Haliotis asinina), decreasing the pH level by 0.19 to 0.57 units relative to normal seawater levels not only decreased hatching rates but also impaired the growth of trocophore larvae. Hatching rates exhibited a significant decline at pH 7.6, which is 0.3 units lower than the normal pH level (Tahil & Dy, 2016). Kimura et al. (2011) also observed notable decrease in hatching rate compared to the control group in H. discus hannai at pH levels ranging from 7.43 to 7.54. This finding aligns with the study conducted by Li et al. (2013) on the same species, which found no significant difference in hatching rate at pH 7.6 to the control group. Substantial differences were observed in the pH 7.3 treatment. These findings suggest that a pH level of 7.54 or lower has a negative impact on the hatching rate of H. discus hannai. However, Pratiwi et al. (2024) discovered that the pH range of 7.0–8.2 had no significant effect on the hatching rate of H. discus hannai. In contrast, H. rufescens and Multicolored abalone (Haliotis diversicolor) exhibited notable variations in hatching rates at pH 7.6 (Gómez-Reyes et al., 2023) and 7.71 (Guo et al., 2015), respectively. Jiang et al. (2021) attributed these decrease in hatching rate to the weakening and loss of elasticity of the egg membrane caused by acidic conditions, resulting in the premature release of the embryo from the egg followed by death. García et al. (2024) suspect that low pH interferes with the ability to regulate acid-base homeostasis in embryos, thus slowing down embryo development and hatching time. Acidification can also induce a stress response in mollusks that enhances energy metabolism for survival, thereby suppressing growth activity (Li et al., 2013). Alternatively, acidic conditions could also decrease RNA concentrations, thereby decreasing the production of the key proteins during early development and lowering hatching rates (Franke & Clemmesen, 2011). Low pH could impact hatching enzyme secretion, decreasing the hatching rate (García et al., 2024).
In some abalone species, lower pH has been linked to deleterious effect on larval growth. Li et al. (2013) reported that H. discus hannai larvae grown at pH 7.6 and 7.3 developed more slowly and exhibited higher deformity rates, slower metamorphosis rates, and a shorter shell length. Pratiwi et al. (2024) also discovered that 30 hpf H. discus hannai larvae cultured at pH 7.0 were significantly shorter than controls. The malformation rate of 30 hpf larvae cultured at pH 7.9, 7.6, 7.3, and 7.0 increased compared to control but was only significant at pH 7.0 and 7.3 (Pratiwi et al., 2024). Similarly, European abalone (Haliotis tuberculata) larvae developed more slowly, had fewer shelled larvae, and were shorter when the pH was 7.7 or 7.6. These abalone larvae also exhibited lower survival and shell mineralization rate (Wessel et al., 2018). When exposed to pH 7.85 and 7.65, the shell length of H. asinina (Linnaeus 1758) shrank and the number of malformations rose compare to the control animals reared under ambient pH conditions (Santander & Sayno, 2018). This decrease in shell length and calcification can occur due to decreased calcium carbonate saturation in more acidic conditions. In turn, the larvae deposit less calcium carbonate and produce a thinner shell. The earliest instance of CaCO3 deposition in abalone occurs during the early veliger stage. During this process, amorphous calcium carbonate (ACC) is deposited and quickly converted into crystalline aragonite (Auzoux-Bordenave et al., 2010). Decreased seawater pH can lead to a lack of saturation of aragonite and calcite, which hinders the generation of new shells or skeletons by larvae (Mostofa et al., 2016). Moreover, decrease in shell size are likely a result of the inhibition of metabolism caused by hypercapnia, leading to delayed development (Brennand et al., 2010). In H. coccoradiata, the impact of pH on the calcification and shell formation process in abalone is further exacerbated by increasing the temperature 2°C and 4°C above normal. Byrne et al. (2011) found that only a minority of eggs developed into healthy larvae under warm temperatures (+2°C to 4°C) and low-pH conditions (pH −0.4 to 0.6 U). Smaller larval mollusks with weaker and thinner shells may require more time in their the planktonic stage to accumulate sufficient energy for metamorphosis. A prolonged larval life span can potentially result in reduced survival rates and elevated mortality rates due to heightened susceptibility to predation and additional environmental stressors (Parker et al., 2013).
The larval settlement process is an essential part of the life cycle of various benthic marine species, including abalone. Recent studies have indicated that future populations of abalone might be adversely affected by a decline in settlement (Espinel-Velasco et al., 2021). Studies investigating the impact of pH on settlement rate have been conducted on many species of H. genus, including Blackfoot paua (Haliotis iris) (Espinel-Velasco et al., 2021), Northern abalone (Haliotis kamtschatkana) (Crim et al., 2011), H. asinina (Tahil & Dy, 2015), European abalone (Haliotis tuberculata) (Kavousi et al., 2022), and H. rufescens (Gómez-Reyes et al., 2023). In H. kamtschatkana, a decrease in pH due to the administration of CO2 did not have a significant impact on the settlement rate (Crim et al., 2011). Similarly, the settlement rate of H. tuberculata at pH 7.7 was not substantially different from the control. Moreover, decreasing the pH to 7.65 did not significantly affect the settlement rate of H. iris (Espinel-Velasco et al., 2021). These result were likely because the examined pH conditions were still within an acceptable range for the species to settle. However, abalones capable of settling under low pH conditions are likely to experience shell damage. In the case of H. kamtschatkana, nearly all larvae that undergo settlement and metamorphosis at a concentration of 1,800 ppm exhibit anomalous shell development or lack a shell altogether. This condition could render them especially susceptible to predation by bottom-dwelling organisms in their natural habitat (Crim et al., 2011). Studies conducted on different abalone species have demonstrated that lower pH levels can negatively impact larval settlement rates. For example, a reduction in pH to 7.76, 7.57, and 7.41 significantly affects the settlement rate of H. asinina (Tahil & Dy, 2015). Gómez-Reyes et al. (2023) found that short-term reduction of pH had a noticeable effect on the settling rates of H. rufescens larvae after being incubated under pH levels of 7.8 and 7.6. The effect of pH on settlement is believed to be caused by the disturbance of abalone chemosensory signals, which hinders their ability to detect appropriate settlement sites under low pH conditions (Crim et al., 2011). Acidic conditions can also disrupt the capacity of crustose coralline algae to cover settlement plates. Tahil & Dy (2015) reported a decrease in the coverage of crustose coralline algae after 15 days of exposure to pH levels of 7.57 and 7.41 compared to the normal pH levels (Tahil & Dy, 2015).
During their early life stages, abalone are more susceptible to the negative impacts of ocean acidification. Therefore, numerous studies have been conducted to characterize the effect of pH on the development of abalone larvae. However, very few studies have analyzed the impact of pH on juvenile and adult abalone. A summary of the impacts of ocean acidification on the juvenile and adult of abalone (Haliotis spp.) is presented in Table 4.
Species | CO2/pH range | Experiment duration | Measured | Findings | References |
---|---|---|---|---|---|
Haliotis laevigata (Juvenile) | pH 9.25, 8.45, 7.95, 7.30, 6.72, and 6.08 | 68 d | Oxygen consumption rate | Reduce at 9.25, 6.72, and 6.08 | Harris et al., 1999 |
H. laevigata (Juvenile) | pH 9.01, 8.27, 7.76, 7.46, 7.16, and 6.79 | 68 d | Survival rate | Reduce at 6.79 | |
Food consumption | Reduce at 7.16 | ||||
Haliotis rubra (Juvenile) | pH 9.01, 8.27, 7.76, 7.46, 7.16, and 6.79 | 68 d | Survival rate | Reduce at 7.76 and 6.79 | |
Food consumption | Reduce at 7.16 | ||||
Haliotis iris (Juvenile) | 450, 1,000, and 1,600 µatm (pH 8.1, 7.8, 7.6) | 100 d in autum/winter and 100 d in spring/summer | Survival | Reduce in large juvenile in pH 7.6 during autumn/winter experiment | Cunningham et al., 2016 |
Shell length | - Shorter at pH 7.6 in autumn/winter for small juveniles - Shorter at pH 7.8 and 7.6 in spring/summer for small juveniles - Shorter at pH 7.8 and 7.6 on autumn/winter for large juvenile |
||||
Growth (wet weight) | - Decreased for small juveniles at both seasons - NS on autumn/winter for large juvenile - Decrease on spring/summer for large juvenile |
||||
Haliotis iris (Juvenile) | 450, 1,000, and 1,600 µatm (pH 8.1, 7.8, 7.6) | 100 d in autum/winter and 100 d in spring/summer | Respiration | NS | Cunningham et al., 2016 |
H. iris (Juvenile) | 400 and 1,000 µatm (pHT 8.00 and 7.66) combine with temperature 13℃ and 15℃ | 4 mon | Survival | NS | Cummings et al., 2019 |
Shell growth | NS | ||||
dry flesh weigh | NS | ||||
Total thickness of the shells | NS | ||||
The prismatic layer | Thinner at low pH | ||||
Nacre layer | Thickened at lowered pH and higher temperature | ||||
Haliotis tuberculata (Adult) | 400 and 1,000 µatm pCO2 (pH 8.0 and 7.7) | 5 mon | Survival rate | NS | Avignon et al., 2020 |
Growth rate | Lower at pH 7.7 | ||||
Haemolymph and muscle weights | NS | ||||
Gonad weight | Decreased weight at lower pH at the end of 4 mon | ||||
Phagocytosis efficiency | NS | ||||
Respiration rate | NS | ||||
Excretion rate | NS | ||||
H. tuberculata (Adult) | 400 and 1,000 µatm pCO2 (pH 8.0 and 7.7). | 5 mon | Calcification related gene expression | NS | Avignon et al., 2020 |
Stress related gene expression | NS | ||||
Shell coloration | Pale at low pH | ||||
Calcification rate | Decrease at low pH | ||||
Shell weight and thickness | NS | ||||
Periostracum thickness | Thinner at low pH | ||||
Shell fracture force | Increase at low pH | ||||
H. tuberculata (Adult) | pH 7.9, 7.7, and 7.4 | 15 d | Survival and growth | NS | Auzoux-Bordenave et al., 2021 |
Haemolymph pHT | Decrease at pH 7.4 | ||||
Total alkalinity (AT) in haemolymph. | Decrease at pH 7.4 | ||||
pCO2 in haemolymph. | Decrease at pH 7.4 in day 10 | ||||
HCO3- hæmolymph | Decrease at pH 7.4 | ||||
Protein content in hæmolymph | NS | ||||
Haliotis diversicolor (Adult) | 560, 880, and 1,600 μatm | 1 year | Shell surface | Pale at low pH and decrease spirorbid density | Guo et al., 2023 |
Haliotis diversicolor (Adult) | 560, 880, and 1,600 μatm | 1 year | Shell growth | Lower in the pCO2 1,600 μatm | Guo et al., 2023 |
Shell thickness | NS | ||||
The ratio of nacre layer thickness to prismatic layer thickness | Higher in the pCO2 1,600 μatm | ||||
Mechanical properties of the shell | Decrease in the pCO2 1,600 μatm | ||||
The number of nano-asperities in the n acre tablet | Decrease in the pCO2 1,600 μatm | ||||
The Na/Ca ratio in the prismatic aragonite layer | Not follow the pattern of increasing with rising pCO2 | ||||
Haliotis discus hannai (Adult) | 560, 880, and 1,600 μatm | 1 year | Shell surface | Periostracum layer pale and decrease spirorbid density at high pCO2 | Guo et al., 2023 |
Shell growth | Decrease only in the pCO2 880 μatm | ||||
Shell thickness | Lower the shell thickness | ||||
The ratio of nacre layer to prismatic layer thickness | NS | ||||
H. discus hannai (Adult) | 560, 880, and 1,600 μatm | 1 year | Mechanical properties of the shell | Decrease in the pCO2 1,600 μatm | Guo et al., 2023 |
The number of nano-asperities in the nacre tablet | NS | ||||
The Na/Ca ratio in the prismatic aragonite layer | NS | ||||
H. discus hannai (Adult) | pH 8.1 and 7.5 combination with 15℃, 20℃, and 25℃ | 5 d | Survival rate | NS | Kim et al., 2023 |
H2O2 and MDA levels in hemolymph | H2O2, MDA levels and SOD, CAT, caspase-3 expression were Increased under low/high temperature and/or low pH | ||||
mRNA expression of SOD enzymes, CAT, and caspase-3 | |||||
Apoptosis level in the hepatopancreas | Higher under high temperatures and low pH conditions | ||||
Haliotis tuberculata (Adult) | pH 8.01 and 7.7 | 3 mon | Food consumption | NS | Roussel et al., 2024 |
Abalone shells consist of two different types of calcium carbonate polymorphs. The outer prismatic layer may be calcite, aragonite, or a combination of both (Cummings et al., 2019), whereas the inner shell (nacre) is made of aragonite (Thompson et al., 2000). Aragonite and calcite saturation may decrease in response to an increase in atmospheric CO2 (Feely et al., 2012), which can influence the development of abalone shells during all life phases from early development to the juvenile and adult phases. Abalone shells provide protection from predators, noxious substances, and adverse environmental circumstances. Therefore, inhibiting abalone shell growth or reducing their mechanical strength may reduce their survival rate (Guo et al., 2022).
Several studies have examined the consequences of ocean acidification on the survival and shell growth of juvenile and adult abalone. Cunningham et al. (2016) investigated the survival and shell growth of both large and small juvenile H. iris. During the experiment, which lasted 100 days in autumn/winter and 100 days in spring/summer, the juveniles were grown at three pH levels (8.1, 7.8, and 7.6). Only large abalone exhibited significant variation in survival rate during the autumn/winter period between pH treatments. However, shell growth exhibited a notable deceleration at low pH levels in both small and larger abalone, regardless of the season. These findings indicate that the impact of pH on the survival rate depend on the size of the juvenile and the season, with the latter parameter being related to the sea water temperature. Particularly, the negative effects of acidification on abalone survical may be exacerbated by higher temperatures (Parker et al., 2013). However, a study conducted by Cummings et al. (2019) demonstrated that exposing juvenile H. iris to a reduced pH of 7.6 for a 4 months did not have any impact on their survival and growth rate. These discrepancies were likely due to difference between the carbonate saturation levels in the aforementioned studies, with the study by Cunningham et al. (2016), reporting a lower saturation, as well as disparities in feed and ambient conditions (Cummings et al., 2019).
The shell growth of juvenile H. tuberculata cultured for three months at four different pH levels (8.1, 7.8, 7.7, and 7.6) was also significantly slower at a lower pH (pH 7.6) compared to the control group. However, survival rates were not markedly different between pH treatments (Auzoux-Bordenave et al., 2020). Similarly, low pH conditions significantly affected juvenile H. discus hannai growth but had no effect on survival rate (Li et al., 2018). In contrast, exposing adult H. tuberculata to three pH levels for 15 days had no significant effect on survival rates and shell growth (Auzoux-Bordenave et al., 2021). These differences were likely attributable to species-specific variations, as well as the duration of the pH treatment. According to Gazeau et al. (2013), the reaction of shelled mollusks to low seawater pH and high pCO2 appears to be species-specific and even varied within species.
The calcification process of abalone shells is known to be negatively impacted by ocean acidification. For example, juvenile H. discus hannai cultivated at pH 7.7 for three months exhibited shell surface deteriorated, with large regions of shell lacking periostracum. Additionally, their brown-colored shell apex was hard to recognize. SEM analysis further confirmed the occurrence of large-scale deterioration of the nacreous layer and periostracum layer in abalone raised at pH 7.7. Calcite dissolution was also noted as exposed nacreous layer surfaces that were uneven and rough (Li et al., 2018). Similarly, adult H. discus hannai and H. diversicolor exposed to high pCO2 conditions for a year exhibited shell periostracum deterioration and reduced resistance to crushing force (Guo et al., 2023). Periostracum alterations were also observed in juvenile H. tuberculata that were cultivated under a pH 7.6. Furthermore, the shell mineral layers exhibited altered texture and porosity (Auzoux-Bordenave et al., 2020). Ocean acidification profoundly affects the thinner prismatic calcite layer, leading to a more noticeable erosion of the outer shell surface of juvenile H. iris at a lower pH of 7.6 (Cummings et al., 2019).
Ocean acidification has also been linked to the erosion and whitening of the periostracum layer, which is likely due to a decrease in the production of sulfated polysaccharides. These polysaccharides play a crucial role in preventing shell degradation (Cummings et al., 2019). Reduced calcification can occur due to either a direct impact on the dissolution of calcium carbonate (CaCO3) or by indirect metabolic influences, such as the regulation of physiological and molecular processes involved in the formation of shell biominerals, including the expression of carbonic anhydrase, chitinase, tyrosinase, and other related factors. The effects of ocean acidification can also vary depending on whether aragonite or calcite are present in the shell, as aragonite is more sensitive to dissolution (Auzoux-Bordenave et al., 2020).
A further impact of thinning the abalone shell is that it can increase the vulnerability of the abalone to predation and pathogens (Barclay et al., 2020). Survival and health are also dependent on its shell because the shell is an external defense system (Muznebin, 2022). Some parasites or pathogens that attack abalone include shell-boring polychaete (Polydora hoplura) (Winkler et al., 2024), shell-boring sponge (Cliona sp.) (Huchette et al., 2006), herpes-like virus (Chang et al., 2005), and spherical viruses (Wang et al., 2004). Chang et al. (2005) first identified the herpes-like virus in 2005, and it was associated with the mass mortality of H. diversicolor supertexta in Taiwan in 2003. Spherical viruses can also cause high mortality and be linked to crack-shell disease (Wang et al., 2004).
Decreases in water pH can lead changes in the immune system (Kim et al., 2023), hemolymph, respiration, and excretion rates (Avignon et al., 2020). Low pH levels, individually and in combination with high temperature stress, lead to oxidative stress in abalone, which can induce an increase in the levels of malondialdehyde (MDA), H2O2, catalase (CAT), superoxide dismutase (SOD), caspase-3, and apoptotic rate in H. discus hannai (Kim et al., 2023). SOD, a main scavenger of O2− formed during stressful situations, inhibits the production of oxygen radicals following exposure to low-pH seawater, stimulating CAT activity (Liao et al., 2019). A decrease in pH, especially when combined with high temperatures, triggers reactive oxygen species (ROS) production, which in turn stimulates the expression of SOD and CAT to stabilize ROS (Kim et al., 2023). Under excessively high ROS conditions, the secreted antioxidant enzymes may not be sufficient to alleviate oxidative stress, leading to DNA damage, enzyme inactivation, and membrane lipid damage (Liao et al., 2019). In turn, increasing cell toxicity can also lead to caspase-3 upregulation, which sends apoptotic signals and leads to cell death (Kim et al., 2023). Another study reported that adult H. tuberculata cultured at pH 7.7 for 1 week, 2 months, and 4 months did not exhibit significant differences in phagocytosis efficiency compared to the control treatment. However, acid-base regulation, as measured by the pHT of hemolymph, was reduced at pH 7.7, leading to general internal acidosis.
Respiration and excretion rates in H. tuberculata also did not vary significantly between pH 8.0 and 7.7 after 4 months (Avignon et al., 2020). Presumably, the 0.3 pH unit drop in seawater pH might have posed a significant challenge for H. tuberculata during the first two months of exposure but the abalones began acclimate after 4 months. In this case, acidification had a greater impact on shell growth and structural integrity than on metabolism (Avignon et al., 2020). However, lowering the pH may also have a significant impact on metabolism in other abalone species. For example, Greenlip abalone (Haliotis laevigata) experienced a decrease in oxygen consumption rate that was significantly different from the control (pH 8.27) after being incubated in seawater with extreme high and extreme low pH (9.25, 6.72, 6.08) for 68 days (Harris et al., 1999). The abalone from this experiment most likely experienced alterations in oxygen-hemocyanin affinity, which is aggravated by anaerobic metabolic products, resulting in a decrease in oxygen consumption rate (Harris et al., 1999).
Reduced seawater pH can influence abalone food consumption; however, the effect varies according to the species and age. At pH 7.16, juvenile H. laevigata consumed considerably less food than at pH 7.76. Meanwhile, juvenile Blacklip abalone (Haliotis rubra) food consumption was significantly reduced at pH 7.16 when compared to pH 9.01 (Harris et al., 1999). Ocean acidification can diminish the ability of mollusks to detect food sources, resulting in a decrease in food consumption (Horwitz et al., 2020). However, there was no significant influence on food consumption of adult H. tuberculata between pH 8.01 and 7.7 after three months of treatment (Roussel et al., 2024).
However, cultivating abalone using formulated feed or seaweed could have negative environmental consequences. Animal waste, including feces and urine, as well as uneaten food, accounts for the majority of waste water from abalone cage farms. This increases the acidity of bottom sediment (Kang et al., 2016), alters benthos populations, and induces eutrophication (Lee et al., 2016). Integrated multi-trophic aquaculture (IMTA) offers an alternate approach to addressing this issue and combating ocean acidification. Hamilton et al. (2022) demonstrate that integrated multitrophic aquaculture has the ability to improve growth, reduce nutrient loads, elevate system pH, and alleviate environmental conditions when compared to standard single-species culture approaches.
Conclusion
The impact of ocean acidification on abalone depends on various aspects, including the species, developmental stage, size, and duration of exposure. Moreover, the effects of pH stress can be compounded with other environmental conditions, thereby having a more pronounced influence on abalone. Therefore, future research should focus on the combined effects of several stressors (e.g., temperature) on the various life-history phases of abalone, as well as the potential for adaptation of acclimatization in certain species.